GC-MS Solutions Enhancing Food and Environmental Testing
Compendium
Published: January 29, 2025
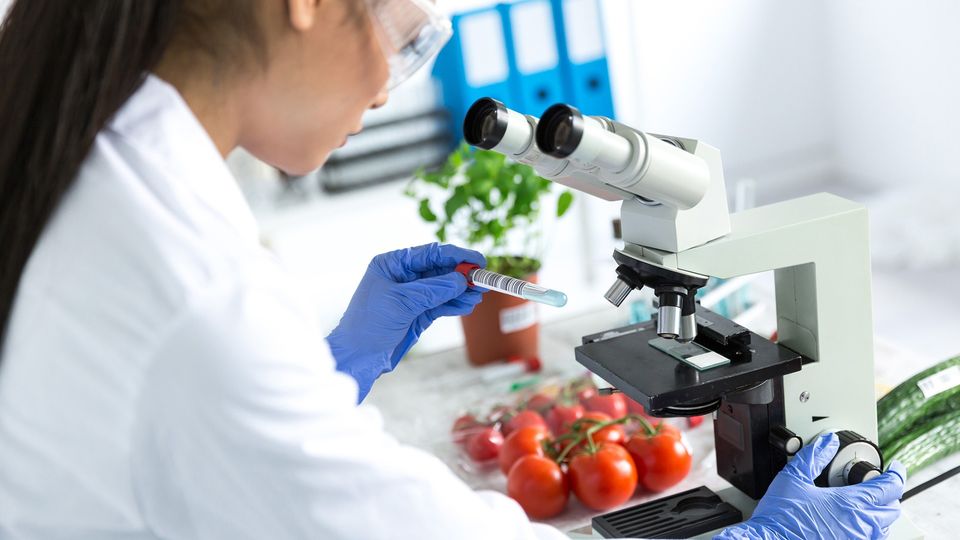
Credit: iStock
Reliable analytical workflows are essential to ensure the safety and quality of our food and environment. However, laboratories often face challenges with precision, sensitivity and time efficiency, especially when analyzing pollutants such as pesticides, PFAS, phthalates and SVOCs.
This compendium offers a collection of application notes highlighting state-of-the-art GC-MS methods that can provide unmatched accuracy, sustainability and productivity for your lab.
Download this compendium to discover:
- Advanced GC-MS solutions for sensitive contaminant detection
- Strategies to achieve high sensitivity and regulatory compliance
- Insights on reducing downtime and enhancing lab efficiency
GC/MS solutions for environmental and food testing
Ensure a Healthier World
from the Ground Up
Application Compendium
Introduction 3
Environmental
Analysis of PFAS and Environmental Contaminants in Soil 4
and Oat Plants with GC/Q-TOF
Analysis of Phthalate with Hydrogen Carrier Gas 18
Automated Sample Prep Using Agilent PAL3 RTC 28
for EPA 8270E SVOC Analysis by GC/TQ
Novel Column Chemistry Raises the Bar on Sensitivity 37
and Data Accuracy in SVOCs Analysis
Accurate Mass Library for PFAS Analysis in Environmental Samples 49
Food
Enhanced Longevity and Revolutionized Robustness for 61
Sensitive Detection of 190 Pesticides Over 800 Injections
Brewing Excellence: Quantitating Over 200 Pesticides in Black Tea 76
with Steady Performance and Maximized Uptime by GC/MS/MS
Essential Oils Analysis Using GC/MS with Hydrogen 101
and the Agilent Hydrolnert Source
Ethylene Oxide and 2-Chloroethanol in Spices and Oilseeds 126
Using QuECHERS GC/MS/MS
Analysis of Aldehydes in Beer by Agilent PAL 3 Autosampler 133
and 5977C GC/MSD
2
Table of Contents
Agilent 5977C GC/MSD Agilent 7000E GC/TQ Agilent 7010D GC/TQ Agilent 7250 GC/Q-TOF
3
Ensuring the safety and quality of our environment and food supply is a significant and evolving
challenge. Analytical testing laboratories are under constant pressure to meet the latest
regulations for contaminants such as pesticides, PFAS, phthalates, and SVOCs. Precise, reliable,
and efficient analytical workflows are paramount to maintaining the safety and quality of our
food and environment.
Agilent GC/MS systems deliver high-quality data, maximize productivity, and minimize
downtime. Built-in instrument intelligence promotes ease-of-use and robust performance, while
sustainability features work to lower your laboratory’s environmental footprint. The application
notes in this compendium demonstrate these advanced GC/MS solutions for the analysis
of water, soil, air, food, and consumer products. See how to meet today's—and prepare for
tomorrow’s—environmental and food testing challenges.
Meet the ever-evolving analytical challenges
for environmental and food testing with
Agilent GC/MS solutions
Application Note
Environmental
Authors
Luann Wong, Gabrielle Black,
and Thomas Young
Department of Civil and
Environmental Engineering,
University of California, Davis
Sofia Nieto, Matthew
Giardina, Matthew Curtis, and
Tarun Anumol
Agilent Technologies, Inc.
Abstract
Soil is one of the major environmental repositories of per- and polyfluoroalkyl
substances (PFAS)1
, and the presence of PFAS in soil can potentially lead
to ground water and food contamination. Current PFAS methods typically
only cover 40 to 80 PFAS and vastly underestimate their presence in many
environmental samples based on mass balance studies.2,3 Further, liquid
chromatography/mass spectrometry (LC/MS) has significant limitations with
respect to the analysis of some of the volatile classes of PFAS, which is where gas
chromatography/mass spectrometry (GC/MS) should be considered as an important
complementary technique.
This study describes different approaches for the extraction and analysis of PFAS in
soil and plants using an Agilent 7250 gas chromatography/quadrupole time-of-flight
mass spectrometer (GC/Q-TOF). PFAS and other environmental contaminants
were detected using a target screening methodology based on an accurate mass
personal compound database and library (PCDL) of these pollutants. A broader
range of contaminants was also identified in the soil and plant samples, including
polychlorinated biphenyls (PCBs), polybrominated diphenyl ethers (PBDEs), and
flame retardants, using a nontargeted screening and an extensive unit mass
NIST23 library.
Analysis of PFAS and Other
Environmental Contaminants in Soil
and Oat Plants Using High-Resolution
GC/Q-TOF
4 Return to Table of Contents
2
Introduction
PFAS are persistent synthetic organic pollutants with a
potential to bioaccumulate.4
The list of PFAS substances
curated by the Environmental Protection Agency (EPA)
currently includes nearly 8,000 PFAS chemicals based on
structure5
ranging from volatile PFAS, such as ubiquitous
fluorotelomer alcohols (FTOHs), to long-chain PFAS, including
the most commonly detected perfluorooctanoic acid (PFOA)
and perfluorooctane sulfonate (PFOS). While long-chain
PFAS are being phased out of production, the manufacturing
of shorter chain length PFAS is increasing due to the
assumption that more volatile PFAS are less toxic. These
shorter chain length PFAS such as 6:2 FTOH are more difficult
to detect with LC/MS using established methods, and recent
studies have indicated that they are equally toxic.6,7
Soil is a significant reservoir of PFAS as well as of many
other persistent environmental contaminants, and thus can
contribute to contamination of ground water, atmosphere,
and biota. Therefore, to better understand the source and
transport of these contaminants, both soil and plant extracts
have been analyzed using the Agilent 7250 GC/Q-TOF.
To maximize the sensitivity of PFAS detection, a target
screening approach based on a PFAS accurate mass library
was used. The PFAS PCDL used in this study included
over 150 electron ionization (EI) PFAS spectra along with
retention times (RTs) and retention indices (RIs), and is
described elsewhere.8
In addition to PFAS, many persistent pollutants were identified
in both soil and plants, where both target and nontarget
screening workflows were used. These pollutants included
pesticides, polyaromatic hydrocarbons (PAHs), PCBs, PBDEs,
and flame retardants.
Experimental
Sample collection
Soil and oat plants were sampled from two fields in California
(F1 and F2) that have historically received biosolids. The soil
samples were collected prior to the application of biosolids
(labeled PreA for preapplication). A certified USDA organic
(Org) field was also sampled prior to treating the subplots
with compost (Comp) and compost and lime (C&L). The
compost was collected as well. The soil was also sampled at
harvest time (Hvst). Plants were collected in the same regions
as the soil samples.
Sample preparation
The soil and plant samples were either extracted with
methylene chloride (DCM) for liquid injections or subjected
to headspace solid-phase microextraction (HS-SPME). For
DCM extraction, 2 g of soil was weighed into a 50 mL glass
centrifuge tube and 5 mL of DCM was added. The 50 mL
centrifuge tubes containing the samples were vortexed using
a Heidolph Multi Reax Vibrating Test Tube Shaker at speed 5
for 5 minutes and centrifuged at 3,000 rpm for 5 minutes.
Approximately 0.5 mL of supernatant extract was transferred
into 2 mL autosampler vials. Whole plant samples that
included stems, leaves, seeds, and seed pods were cut into 2
to 5 mm sections. Then, 2 g of plant samples were extracted
with methylene chloride the same way as the soil. Method
blank samples for each set were also generated.
For HS-SPME, the soil (2 g) and finely chopped plant material
(1 g) were transferred into a 20 mL headspace vial, and either
2 or 3 mL of DI water were added, respectively.
SPME conditions
The HS-SPME was performed using an Agilent PAL 3 CTC
autosampler. Four different fibers were tested (Agilent 100 µm
PDMS, 95 µm CWR/PDMS, 65 µm DVB/PDMS, and 80 µm
DVB/CWR/PDMS, part number 5191-5878), and the SPME
conditions were optimized. The fiber conditioning was carried
out at 300 °C for 5 minutes. The samples were equilibrated
for 10 minutes, and the SPME fiber was inserted into the vial
headspace. Extraction was carried out at 50 °C for 35 minutes
at 300 rpm (programmed for 10 seconds on, 2 seconds
off cycle), with the desorption into the GC inlet at 250 °C
for 7 minutes. The GC injection port was equipped with the
0.75 mm id liner for SPME analysis and the resistant to wear
Merlin Microseal septa.
5
3
Data acquisition
The GC/MS analysis was performed using an Agilent 7250
GC/Q-TOF system. All the data were acquired in full spectrum
acquisition mode. Two different GC columns were used to
acquire the data. The DB-624 is a midpolar GC column and
provided the best retention and separation for GC-amenable
PFAS compounds. This column was used for PFAS screening
using the PFAS PCDL. The nonpolar DB-5ms column was also
used to take advantage of RI information available for all the
compounds with EI spectra in the NIST23 library. The data
acquisition parameters are described in Table 1.
Table 1. Data acquisition parameters.
Agilent DB-5ms Agilent DB-624
MS Agilent 7250 GC/Q-TOF
GC Agilent 8890 GC
Inlet Multimode inlet, Agilent Ultra Inert 4-mm liner single taper
with wool
Inlet Temperature 70 °C for 0.01 min,
300 °C/min to 250 °C
Injection Volume 1 µL
Column
Agilent J&W DB-5ms Ultra
Inert (UI), 30 m × 0.25 mm,
0.25 µm
Agilent DB-624 Ultra Inert,
30 m × 0.25 mm, 1.4 µm
Oven Temperature
Program
35 °C for 2 min,
7 °C/min to 210 °C,
20 °C/min to 300 °C,
4 min hold
30 °C for 2 min,
3 °C/min to 75 °C,
2 °C/min to 110 °C,
10 °C/min to 210 °C,
20 °C/min to 240 °C,
2 min hold
Column Flow 1.2 mL/min constant flow 1 mL/min constant flow
Carrier Gas Helium
Transfer Line
Temperature
250 °C
Quadrupole
Temperature
150 °C
Source Temperature 200 °C
Electron Energy 70 eV
Emission Current Variable by time segment, 0.01 to 5 µA
Spectral Acquisition
Rate
5 Hz
Mass Range (Tune) 50 to 1,200 m/z
Data processing
The nontargeted workflow was performed in
Agilent MassHunter Unknowns Analysis software
(version 12.1) and involved the SureMass chromatographic
deconvolution and the NIST23 EI library search. RIs and
accurate mass information were used to confirm the
compound identification. The suspect screening was
performed using the GC/Q-TOF Screener tool of MassHunter
Quantitative Analysis software (version 12.1) and accurate
mass libraries for pesticides and PFAS.
Results and discussion
Characteristic EI fragmentation of PFAS
One of the approaches that could be beneficial for PFAS
screening in complex matrices is a suspect screening
approach since it allows for high sensitivity and specificity
of detection. When using a high-resolution accurate mass
GC/MS, this approach can be greatly facilitated by using
accurate mass libraries to screen for a large number of
target compounds that could, in theory, be unlimited. Thus,
the accurate mass GC/MS PCDL for over 100 volatile and
semivolatile PFAS compounds that was previously created6
was used in this work for PFAS screening in soil and plant
samples. The PFAS compound classes in the PCDL included
perfluoroalkyl iodides (PFAIs), fluorotelomer iodides (FTIs),
fluorotelomer alcohols (FTOHs), fluorotelomer olefins
(FTOs), fluorotelomer acrylates (FTACs), fluorotelomer
methacrylates (FTMACs), fluorotelomer carboxylic acids
(FTCA), fluorotelomer unsaturated carboxylic acids (FTUCA),
perfluoroalkane sulfonamides (FASA), among others, many of
which are uniquely amenable to GC/MS analysis. The electron
ionization (EI) mode was chosen for the PFAS PCDL as a
more universal technique compared to chemical ionization.
EI covers a broader range of PFAS compound classes and
allows users to easily screen for other contaminants in the
same data file. While many PFAS compounds can highly
fragment in EI, most of them nevertheless have specific
fragment ions that could be selected by the GC/Q-TOF
suspect screening algorithm or manually, as target or qualifier
ions. Some of the most typical and specific fragments for the
different PFAS compound classes are shown in Table 2.
6
4
Characteristic
Fragments
Neutral Loss
(m/z)
PFAS Class; % of Base Ion, as Maximum Observed for a Given PFAS Class
FTOH PFAI FTI FTAC FTMAC FTO PFAL FASA
[M]+ 0 – 40 100 30 90 – – –
[M-I]+ 126.9045 – 100 – – – – – –
[M-H2
O-HF]+ 38.0168 100 – – – – – – –
[M-CHO-F]+ 48.011 – – – – – – 90 –
[M-H2
O-F-HF-C2
H2
]+ 83.0308 80 – – – – – – –
[M-H2
O-2F]+ 56.0074 70 – – – – – – –
[M-C2
F5
]+ 118.992 – – 50 – – – – –
[M-HF-I]+ 146.9107 – – 50 – – – – –
[M-H2
O-CF3
]+ 87.0058 30 – – – – – – –
[M-H2
O-2F-CF3
]+ 126.0026 30 – – – – – – –
[M-F]+ 18.9984 6 – – 10 5 5 – 1
[M-CHO-2F]+ 66.9995 – – – – – – 25
[M-SO2
-CH3
]+ 78.9854 – – – – – – – 25
[M-H2
O-CF2
]+ 68.0074 25 – – – – – – –
[M-HF]+ 20.0062 20 – – – – – – –
[M-2F-CF3
]+ 106.992 – – – – – 20 – –
[M-H]+ 1.0078 15 – 1 – – – – –
[M-CH3
]+ 15.0235 – – – – 10 – – 5
[M-H-HF]+ 21.0141 15 – – – – – – –
[M-F-2HF]+ 59.0109 15 – – – – – – –
[M-H2
O-F]+ 37.009 15 – – – – – – –
[M-CF3
-HF]+ 89.0014 – – 10 – – 5 – –
[M-F-HF]+ 39.0046 – – – – – 10 – –
[M-NH2
SO2
]+ 79.9806 – – – – – – – 10
[M-C2
H3
-2F]+ 65.0203 – – – – – 10 – –
[M-CHO]+ 29.0027 – – – – – – 5 –
[M-SO2
-H]+ 64.9697 – – – – – – – 5
[M-SO2
-F]+ 82.9603 – – – – – – – 5
[M-SO2
-CF3
-HF]+ 152.9633 – – – – – – – 5
Table 2. Characteristic fragments of volatile PFAS in EI.
7
5
Selection of SPME fiber for soil analysis
Four different SPME fibers were evaluated for the ability
to extract volatile compounds (including PFAS) from soil:
PDMS, CWR/PDMS, DVB/PDMS, and DVB/CWR/PDMS. The
test was performed using soil (2 g) sampled from the same
location, mixed with 2 mL of water, and run under the same
SPME conditions. Total ion chromatograms (TIC) generated
by each fiber tested are shown in Figure 1. Both DVB/PDMS
and DVB/CWR/PDMS fibers produced a significant number
of peaks and showed the ability to extract a wide range
of compounds.
The number of the identifiable peaks was also evaluated for
each of the fibers (Table 3). DVB/PDMS and DVB/CWR/PDMS
had a comparable number of library hits that was slightly
higher for DVB/CWR/PDMS. Therefore, it was selected for
further analysis.
Table 3. SPME fibers performance. The number of components generated
by the SureMass deconvolution algorithm as well as number of NIST23
library hits (Library Match Score cutoff 75) are shown.
Fiber Type Number of Components Number of Hits
PDMS 422 228
CWR-PDMS 514 419
DVB-PDMS 687 560
DVB-CWR-PDMS 683 570
0
2.5
5.0
7.5
0
2
4
6
0
2
4
0
2
4
2 4 6 8 10 12 14 16 18 20 22 24 26 28 30 32 34 36 38 40 42 44 46
PDMS 100 µm
CWR-PDMS 95 µm
DVB-PDMS 65 µm
DVB-CWR-PDMS 80 µm
×107
×107
×107
×107
Acquisition time (min)
Counts Counts Counts Counts
Figure 1. SPME fiber performance on soil samples.
8
6
Detection of volatile PFAS in soil and plant samples using
accurate mass PFAS library
For PFAS detection using the accurate mass PFAS PCDL for
GC/Q-TOF, the midpolar DB-624 GC column (for more detail,
see Table 1) was used.
Both targeted and nontargeted methodologies that involved
the GC/Q-TOF Screener and the Unknowns Analysis
software, respectively, were used to identify PFAS in soil
and plant samples. An advantage of using the nontargeted
analysis is that both accurate mass libraries, as well as
large comprehensive public libraries such as NIST, could
be used to screen for the contaminants simultaneously
without reinjection.
There are several benefits of the targeted, PCDL-based
suspect screening approach, which is performed entirely
in MassHunter Quantitative Analysis software and has
been described in detail previously.9,10 Some of the main
advantages of this approach include high sensitivity and a
high degree of flexibility and automation of the data analysis
method setup and results validation. The validation requires
minimal human involvement prior to the reporting. Together
this provides a user with a highly effective and time-saving
tool for targeted analysis.
A few PFAS compounds were detected when analyzing the
data from SPME. An example of a compound identified
in a few soil and plant samples using the GC/Q-TOF
Screener is shown in Figure 2. This compound is a
volatile 6:2 fluorotelomer alcohol, frequently detected in
environmental matrices. Due to the trace amount of this
compound, it was not found in a nontargeted approach.
Figure 2. 6:2 FTOH detected in soil using SPME and PFAS PCDL-based screening approach. The mirror plot at the top shows the deconvoluted compound
spectrum versus the spectrum from the PFAS PCDL. The mirror plot at bottom displays only target and qualifier ions.
Sample: soil, F1
9
7
When performing nontarget analysis, the chromatographic
deconvolution uses the SureMass algorithm, which is
specifically optimized for high-resolution EI data to ensure
high speed, sensitivity, and integrity of spectral extraction.
While the suspect screening approach provided the highest
degree of sensitivity and was able to detect more PFAS
compounds, one of the more abundant PFAS was detected in
both approaches (Figures 3A and 3B).
All the PFAS compounds identified in soil and plant samples
were detected by matching the PFAS PCDL.
Figure 3. PFAS (ethyl perfluorobutyl ether) identified in DCM soil extracts using PFAS PCDL in both target screening (A) and an untargeted, deconvolution-based
approach in the Unknowns Analysis software (B).
A Sample: soil, PreA F1
B Sample: soil, PreA F1
10
8
Overall, the HS-SPME approach for PFAS extraction worked
best and provided a higher number of identified volatile PFAS
compounds. The amounts of PFAS detected (summarized
in Table 4) were estimated based on the standard injections
where the actual concentrations in soil and plant samples
have not been determined.
Identification of other contaminants in soil and oat plants
The identification of the additional contaminants in soil and
plant samples was performed for both DCM extracts and
SPME. However, while SPME allowed better detection of
volatile compounds, a higher number of the environmental
contaminants that included PCBs, PBDEs, pesticides, PAHs,
and flame retardants, were detected in DCM extracts and will
thus be the focus of the further discussion.
The separation was carried out using the DB-5ms UI
column to be able to use the RI values from the extensive
NIST23 library, and thus increase confidence in compound
identification by using the RI penalty function for library hits.
This column phase is also compatible with the GC/Q-TOF
Pesticide PCDL, which could be considered for screening
GC/Q-TOF accurate mass EI data for pesticides and PAHs.
After a quick prescreening, the identified pollutants were
grouped by contaminant classes and approached separately.
PCBs and PBDEs were identified in the nontargeted approach
using the Unknowns Analysis and NIST23 library. To eliminate
false positives based on the accurate mass EI data while
searching a unit mass library, the Unknowns Analysis
ExactMass tool was used. This tool is described in further
detail in Figure 4A, which shows an example of one of the
BDEs detected in a soil extract.
There were 20 different PCBs and PBDEs detected in the soil
extracts (Figure 4B). The only oat plant extract where this
group of contaminants (BDE-47) was detected was grown in
field F2.
Note that PCBs and PBDEs were not detected by SPME due
to their high boiling point.
Another prominent group of contaminants identified in
soil extracts was pesticides, and the GC/Q-TOF Screener
workflow with Pesticide PCDL was used for quick and
streamlined detection of these pollutants. The original version
of the Pesticide PCDL, which is RT-based, was supplemented
with RIs to be able to use the PCDL in the screener
workflow together with the data acquired using a different
chromatographic method. The GC/Q-TOF Screener method
was set up in agreement with the SANTE guidelines. However,
RT windows were expanded to allow for an additional RT error
introduced using a different chromatographic method.
Table 4. PFAS detected in soil and plants by HS-SPME using the accurate mass PFAS PCDL and suspect screening approach. The estimated amounts (in pg
on column) are shown.
Compound RT
Quantifier
Ion
Soil Samples Plant Samples
F1 PreA
F1
Hvst
F2
Hvst
C&L
Hvst
Compost
Hvst
Organic
Hvst
Organic
Compost F1 F2 Compost C&L Org
Ethyl Perfluorobutyl Ether 4.4 218.9851 150.2 – – – – – – – – – – –
6:1 Fluorotelomer Alcohol 20.94 130.9915 – 2 – – – – – 2.2 – – – –
6:2 Fluorotelomer Alcohol 23.59 296.0054 – 7.5 0.3 – – – 6.9 2.5 – – – –
N-Methylperfluorooctanesulfonamide 43.1 93.9957 0.3 3.4 0.9 2.1 0.4 1.2 0.9 0.2 – – – –
11
9
Figure 4. PCBs and PBDEs in soil DCM extracts using NIST23. (A) An example of BDE detected in soil sample collected from F1 at the time of harvesting.
ExactMass table (bottom-left panel) shows how well the accurate mass fragment ions matched the unit mass library hit and thus provides the additional
confirmation of compound ID. The most selective and abundant ions are highlighted in the mirror plot when m/z corresponds to the library hit formula. The arrow
in the component chromatogram points to the identified component EICs. (B) Bar graph showing responses of PCB and PBDE for all the soil samples where they
have been identified.
A Sample: soil, F1
B
BDE-99
0
50,000
100,000
150,000
200,000
250,000
300,000
Area
BDE 154
BDE 99
BDE 100
PCB 194
BDE 47
PCB 170
PBDE 71
PCB 171
PCB 183
PCB 128
PCB 177
PCB 153
PCB 146
PCB 123
PCB 149
PCB 151
PCB 122
PCB 125
PCB 98
PCB 11
F1 (PreA) F1 (Hvst) F2 (Hvst) Compost Compost
(Hvst)
CL (Hvst) Org (Hvst)
12
10
Since the DCM extraction method was not optimized
specifically for pesticide recovery from plant matrices, only
soil samples were processed. In total, over 50 pesticides were
detected in soil extracts (Figure 5 and Table 5).
A large number of pesticides were detected in compost
and compost-treated soils, and a few pesticides were
also identified in organic soil extracts. Another interesting
observation was that the insecticides fipronil sulfide and
fipronil sulfone were consistently found in the same soil
samples. Also, conazole fungicides such as propiconazoles,
myclobutanil and difenconazole were mostly detected in
compost and compost-treated soils.
Table 5. Pesticides detected in soil extract using the accurate mass Pesticide PCDL and suspect screening approach.
Compound Name RT RT Delta*
Library Match
Score F1 PreA F1 Hvst F2 Hvst C&L Hvst Compost Hvst Org Hvst Org Compost
1,2,4-Trichlorobenzene 14.62 0.17 98.1 x
Diuron Metabolite 16.56 0.28 99.9 x x x x x x
1,2,3,5-Tetrachlorobenzene 16.98 0.32 90.7 x
2,4,6-TCP/2,4,6-Trichlorophenol 17.44 0.25 99.3 x x x Trace
Nicotine 17.56 0.05 97.9 x
Lufenuron 18.71 0.22 99.7 x x
3,4-DCA/3,4-Dichloroaniline 18.88 0.21 99.9 x x x
Pentachlorobenzene 20.42 0.35 99.4 x x x
DEET/Diethyltoluamide 21.46 0.22 82.1 Trace Trace Trace x x Trace x
2,3,4,5-Tetrachloroanisole 22.74 0.32 99.8 x x
Bromoxynil 23.14 0.09 99.9 Trace x x
HCB/Hexachlorobenzene 23.52 0.36 99.7 x x x Trace x x x
Dichloran (Dicloran) 23.84 0.16 97.8 x x x
Swep (MCC) 24.27 0.10 85.6 x x x
PCP/Pentachlorophenol 24.27 0.24 99.7 Trace x x
Figure 5. GC/Q-TOF Screener window of MassHunter Quantitative Analysis software when screening for pesticides in soil extracts using the Pesticides PCDL.
Sample: soil, F1
13
11
Compound Name RT RT Delta*
Library Match
Score F1 PreA F1 Hvst F2 Hvst C&L Hvst Compost Hvst Org Hvst Org Compost
Pyrimethanil 25 0.10 82.5 Trace x
Chlordene 25.1 0.03 98.5 x
Pentachloroaniline 25.74 0.24 99.7 x x x x x
Dithiopyr 26.85 0.40 93.7 x x x x x x
Anthraquinone 27.59 0.05 99.7 x x x x x x x
4,4'-Dichlorobenzophenone 27.86 0.02 80.3 x x
Fipronil Sulfide 28.13 0.43 99.7 x Trace x x
Cyprodinil 28.27 0.05 99.1 Trace x x
Diuron 28.28 0.31 78.1 Trace x Trace
Fluopyram 28.49 0.18 93 x x x
Chlorbenside 28.99 0.21 92.2 x x x
Chlordane-trans (γ-Chlordan) 28.82 0.03 99.7 x x x x x x x
Triclosan 28.85 0.03 99.3 x x x x x
Chlordane-cis (α-Chlordan) 29.06 0.04 99.9 x x x x x x x
Nonachlor-trans 29.11 0.07 99.9 x x x x x x x
Flutolanil 29.23 0.11 85.2 x x
Fludioxonil 29.27 0.18 99.6 x
Dieldrin 29.5 0.05 84.6 x Trace Trace
p,p'-DDE 29.42 0.04 99.2 x x x x x x x
Oxadiazon 29.43 0.14 97.7 x x x
o,p'-DDD (Mitotane) 29.52 0.07 99.9 x x x Trace x x
Fipronil Sulfone 29.34 0.33 98.6 Trace x Trace x x
Myclobutanil 29.48 0.11 98.8 x x
p,p'-DDD 30.03 0.02 99.5 x x x x x x Trace
Nonachlor-cis 30.03 0.04 99.9 x x x Trace Trace x
Carfentrazone-ethyl 30.32 0.13 98.8 x
Bromoxynil octanoate 30.39 0.06 88.4 x x Trace
Propiconazole I 30.43 0.11 96.4 x x Trace x
Chloridazon (PAC) 30.44 0.06 91.5 x
Propiconazole II 30.5 0.04 96 x x Trace x
Tebuconazole 30.7 0.03 92.7 x x x
Chlorbenside Sulfone 30.7 0.39 89.6 Trace Trace x x x
Bifenthrin 31.08 0.09 98.6 Trace x Trace x x x x
cis-Permethrin 32.19 0.00 99.1 x x x Trace x x x
trans-Permethrin 32.28 0.01 81 x Trace Trace
Difenconazole II 34.09 0.01 88.9 Trace x
* RT delta is recalculated from RI.
Trace indicates that the Library Match Factor is below 75.
14
12
PAHs are included into the Pesticide PCDL and were screened
together with pesticides in a single workflow. PAHs were
mostly detected in soil extracts. However, phenanthrene and
fluoranthene were also identified in most plant samples.
This was not unexpected considering that phenanthrene
and fluoranthene were the most abundant PAHs detected in
the soil.
A prominent group of contaminants identified in soil and oat
plants was flame retardants. The accurate mass spectra
for most of these compounds are already included in the
Pesticide PCDL. For a more comprehensive coverage of this
group of pollutants, a couple of flame retardants identified
in the Unknowns Analysis with NIST23 library, missing in the
Pesticide PCDL, were added to the Quant method directly
from the Unknowns Analysis software, and thus were
screened together with the rest of the targets. Remarkably
similar responses were observed between soil and plant
extracts for this group of pollutants (Figure 7).
Among the most abundant flame retardants identified
in soil and plant samples were tributyl phosphate,
tris(2-chloropropyl) phosphate, and tris(3-chloropropyl)
phosphate, the phosphorus flame retardants of frequent use.
0
500,000
1,000,000
1,500,000
2,000,000
2,500,000
Area
0
5,000,000
10,000,000
15,000,000
20,000,000
25,000,000
30,000,000
35,000,000 Benzo[ghi]perylene
Dibenz[a,h]anthracene
Benzo[a]pyrene
Benzo[k]fluoranthene
Benz[a]anthracene
Fluoranthene
Anthracene
Phenanthrene
Fluorene
Acenaphthene
Acenaphthylene
F1 (PreA) F1 (Hvst) F2 (Hvst) Compost Compost Naphthalene
(Hvst)
CL (Hvst) Org (Hvst)
Figure 6. PAHs detected in soil DCM extracts using the accurate mass Pesticide PCDL and suspect screening approach. The bar graph shows the PAH peak area.
15
13
Conclusion
PFAS analysis in environmental matrices is a challenging
undertaking. In this application note, different approaches for
PFAS extraction from soil and plants as well as downstream
workflows of data processing have been discussed. The most
efficient and sensitive approach for volatile PFAS analysis in
soil and plants suggested here is HS-SPME combined with
the suspect screening based on the PFAS accurate mass
library and high-resolution accurate mass GC/Q-TOF.
In addition, both soil and plant extracts were screened for
other contaminants, and various pollutants including PCBs,
PBDEs, PAHs, pesticides, and flame retardants were identified
using targeted, nontargeted, and combined methodologies.
Figure 7. Flame retardants detected in soil and plant DCM extracts using a combined screening approach that included both accurate mass PCDL as well as
NIST23 library. The bar graph shows flame retardant response in soil (S) and plant (P) extracts.
S
S
P
S
P
S
S
P S
P
S
P
0
100,000
200,000
300,000
400,000
500,000
600,000
700,000
Area
2-Ethylhexyl diphenylphosphate (octicizer)
TPP/Triphenyl phosphate
Bis(3-chloro-1-propyl)(1-chloro-2-propyl)phosphate
Tris(3-chloropropyl)phosphate
TCPP/Tris(2-chloropropyl) phosphate
Tri(2-chloroethyl) phosphate
TBP/Tributylphosphate
2,4,6-Tribromophenol
2,4,6-Tribromoanisole
F1 (PreA) F1 (Hvst) F2 (Hvst) Compost Compost
(Hvst)
CL (Hvst) Org (Hvst)
16
www.agilent.com
DE45375629
This information is subject to change without notice.
© Agilent Technologies, Inc. 2024
Printed in the USA, May 6, 2024
5994-7351EN
References
1. Brusseau, M. L.; Anderson, R. H.; Guo, B. PFAS
Concentrations in Soils: Background Levels versus
Contaminated Sites. Sci. Total Environ. 2020, Oct 20; 740,
140017. DOI: 10.1016/j.scitotenv.2020.140017
2. Lin, H.; Taniyasu, S.; Yamazaki, E.; Wu, N.; Lam, P. K. S.;
Eun, H.; Yamashita, N. Fluorine Mass Balance Analysis
and Per- and Polyfluoroalkyl Substances in the
Atmosphere. J. Hazard. Mater. 2022, Apr 28; 435, 129025.
DOI: 0.1016/j.jhazmat.2022.129025
3. Spaan, K.; Van Noordenburg, C.; Plassman, M.;
Schultes, L.; Shaw, S. D.; Berge,r M.; Heide-Jørgensen,
M. P.; Rosing-Asvid, A.; Granquist, S.; Dietz, R.; et al.
Fluorine Mass Balance and Suspect Screening in Marine
Mammals from the Northern Hemisphere. Environ. Sci.
Technol. 2020, Mar 12, 54(7), 4046–4058. DOI: 10.1021/
acs.est.9b06773
4. Schildroth, S.; Rodgers, K. M.; Stynar, M.; McCord, J.;
Poma, G.; Covaci, A.; Dodson, R. E. Per-and Polyfluoroalkyl
Substances (PFAS) and Persistent Chemical Mixtures in
Dust from U.S. Colleges. Environ. Res. 2021, Apr 15, 206,
112530. DOI: 10.1016/j.envres.2021.112530
5. Williams, A. J; Gaines, L. G. T.; Grulke, C. M.; Lowe, C. N.;
Sinclair, G. F. B.; Samano, V.; Thillainadarajah, I.; Meyer, B.;
Patlewicz, G.; et al. Assembly and Curation of Lists of
Per- and Polyfluoroalkyl Substances (PFAS) to Support
Environmental Science Research. Front. Environ. Sci.
2022, Apr 5, 10, 1–13. DOI: 10.3389/fenvs.2022.850019
6. Sunderland, E. M.; HuHu, X. C.; Dassuncao, C.;
Tokranov, A. K.; Wagner, C. C.; Allen, J. G. A Review of the
Pathways of Human Exposure to Poly- and Perfluoroalkyl
Substances (PFASs) and Present Understanding of Health
Effects. J. Expo. Sci. Environ. Epidemiol. 2019 Mar 29, (2),
131–147. DOI: 10.1038/s41370-018-0094-1
7. Rice, P. A.; HuAungst, J.; Cooper, J.; Bandele, O.;
Kabadi, S. V. A Comparative Analysis of the Toxicological
Databases for 6:2 Fluorotelomer Alcohol (6:2 FTOH) and
Perfluorohexanoic Acid (PFHxA). Food Chem. Toxicol.
2020 Apr, 138, 111210. DOI: 10.1016/j.fct.2020.111210
8. Wong, L.; Black, G.; Young, T.; Nieto, S. Accurate Mass
Library for PFAS Analysis in Environmental Samples and
Workflow for Identification of Pollutants in Drinking Water
Using GC/Q-TOF. Agilent Technologies application note,
publication number 5994-6966EN, 2023.
9. Van Gansbeke, W.; Albertsdóttir, A. D.; Polet, M.;
Van Eenoo, P.; Nieto, S. Introducing Semi-Automated
GC/Q-TOF Screening with the AssayMAP Bravo Sample
Prep Platform for Antidoping Control. Agilent Technologies
application note, publication number 5994-6702EN, 2023.
Application Note
Environmental
Authors
Dinh Loc Dao,
Minh Trung Tran,
Xuan Dai Phan,
Quoc Trung Pham,
Thi Linh Nguyen, and
Tuan Dat Ho
IndoChina Center of
Excellence and
Red Star Vietnam Company
Limited – CMS.
Ho Chi Minh City, Vietnam
Boonraksa Srisawang
Agilent Technologies, Inc.
Singapore, Singapore
Abstract
Gas chromatography/mass spectrometry (GC/MS) plays a pivotal role in the
analysis of phthalates in consumer products. In light of the recent helium supply
challenges, the adoption of hydrogen (H2
) as a carrier gas has gained prominence.
However, using hydrogen as carrier gas in many GC/MS analyses may cause
hydrogenation or dechlorination problems in the ion sources that use helium gas as
carrier gas. The Agilent GC 8890, coupled with the Agilent 5977C GC/MSD and using
the Agilent HydroInert source with hydrogen as the carrier gas with the backflush
technique, provides an effective solution to these challenges. It not only meets
International Electrotechnical Commission (IEC) requirements but also successfully
addresses the issue of instability when dealing with complex sample matrices.
Analysis of Phthalate with
Hydrogen Carrier Gas
Using the Agilent HydroInert source
on a challenging cable sample in gas
chromatography/mass spectrometry
18 Return to Table of Contents
2
Introduction
Phthalates are a group of chemical compounds that are
synthesized from phthalic acid. They are widely used as
plasticizers in many products, including children's toys and
electrical and electronic equipment. Unfortunately, phthalates
can leach out of plastic materials and enter the environment.
This exposure can have adverse effects on human health.
The prevalence of phthalates in various products and the
associated health risks have led to the implementation
of regulations governing their use. Relevant policies
include the European Union’s updated directive under the
Restriction of Hazardous Substances (RoHS) framework,
designated as 2015/863/EU1
, and its 2007 Registration,
Evaluation, Authorization, and Restriction of Chemicals
(REACH) framework.2
Traditionally, helium has been the preferred carrier gas for
GC/MS analysis. However, due to recurring helium shortages
and rising costs, hydrogen is emerging as a viable alternative.
Hydrogen is more cost-effective and efficient than helium,
making it a promising prospect for phthalate analyses.
This application note centers on the analysis of phthalates
using a GC/MS system in Selected Ion Monitoring (SIM)
mode with hydrogen serving as the GC carrier gas. When
transitioning to hydrogen for GC/MS analysis, several critical
factors must be considered.
Hydrogen, being a chemically reactive gas, can induce
chemical reactions within various components of the
GC/MS system, including the inlet, column, and sometimes
the mass spectrometer Electron Ionization (MS EI) source.
These reactions can alter the mass spectra of peaks in
the total ion chromatogram (TIC), potentially leading to the
misidentification of compounds in the analysis results.
The Agilent HydroInert source was developed to mitigate
these concerns related to the MS source. The HydroInert
source, when used with hydrogen as the carrier gas,
preserves mass spectral accuracy and allows users to
continue using existing helium-based mass spectral libraries
and quantitative methods.
This application note uses the solvent extraction method,
which is in accordance with the analytical methodology
outlined in IEC standard 62321-8:2017.3
The presence of
residual sample matrix components in this method can
contaminate essential column components, such as the
inlet, column, and ionization source. This contamination can
adversely affect the accuracy and reliability of the instrument
during analysis.
The self-cleaning capability of the hydrogen gas ionization
source, combined with the backflush technique, helps
address issues related to sample background effects, reduces
maintenance frequency, and ensures precision and accuracy
throughout the analysis process.
Experimental
Reagents and chemicals
– Tetrahydrofuran (THF) 99.9% was purchased from
Sigma-Aldrich.
– HPLC-grade acetonitrile (ACN) was obtained from Supelco.
– Benzyl benzoate (BB) 98%, benzyl butyl phthalate (BBP)
98%, dibutyl phthalate (DBP) 98%, di-isobutyl phthalate
(DIBP) 98%, di-n-pentyl phthalate (DPENP) 2,500 mg/L,
di-n-hexyl phthalate (DHEXP), dicyclohexyl phthalate
(DCHP) > 98%, di(2-ethylhexyl) phthalate (DEHP) > 98%,
dinoctyl phthalate (DNOP) > 98%, di-isononyl phthalate
(DINP) > 98%, and di-isodecyl phthalate (DIDP) were
obtained from Sigma-Aldrich.
Standards solution and standards preparation
Phthalate ester standard solutions were prepared by diluting
a mixture of phthalate ester standards at a concentration
of 100 mg/L in a mixture of THF:ACN (1:1) to create a
series of standards with concentrations of 0.2, 0.5, 1, 2, and
5 µg/mL, respectively. Also, each of the standard solutions
was supplemented with an internal standard, BB, at a
concentration of 1 µg/mL.
Sample preparation
1. Weigh 300 mg of the sample and transfer it to a 40 mL
vial. Record the mass to the nearest 0.1 mg.
2. Add 10 mL of THF and 30 µL of internal standard BB
(1,000 µg/mL) to the vial.
3. Seal the vial tightly and place it in an ultrasonic bath.
Sonicate for 30 to 60 minutes until the sample dissolves.
4. After the sample has completely dissolved, let the vial cool
to room temperature.
5. Carefully add 20 mL of ACN drop by drop into the vial to
precipitate the sample matrix.
6. Let the resulting solution stand at room temperature for
30 minutes.
7. Allow the polymer to settle or filter the mixture through a
0.45 µm polytetrafluoroethylene membrane.
8. Inject on GC/MS.
19
3
Instrumentation
The experimental system used in this study was
configured (Figure 1) to address and reduce potential
challenges arising from the use of hydrogen as the
carrier gas and the complexity of the sample matrix in
phthalates analysis. The system comprised an 8890 GC
configured with an Agilent 7693A automatic liquid sampler
(G4513A) and a split/splitless inlet coupled to a 5977C
gas chromatography/mass selective detector (GC/MSD)
configured with a HydroInert source.
Tables 1 and 2 show the GC and MS conditions for
phthalate analysis and the SIM ion parameters used for
data acquisition.
Parameter Value
Agilent 8890 GC
SSL Inlet 250 °C, Mode: split (5:1)
Liner Inlet liner, Ultra Inert, split, low pressure drop,
glass wool (p/n 5190-2295)
Injection Volume 1 µL
Column 0.7 mL/min, constant flow mode (H2
) Agilent DB-5ms Ultra
Inert, 30 m × 0.18 mm, 0.18 µm (p/n 121-5522UI)
Restrictor
1.1 mL/min, constant flow mode (H2
)
Purge flow: 3 mL/min
Agilent DB-5ms Ultra Inert 5 m × 0.15 mm, 0.15 µm
(p/n 165-6626)
Oven Program
110 °C (0.5 min)
30.5 °C/min to 280 °C (0.5 min)
30.5 °C/min to 320 °C (2 min)
Agilent 8890 GC Backflush Parameters
Inlet Pressure
(Backflushing) 2 psi
Backflush Pressure 20 psi
Void Volumes 5.1715
Backflush Time 4 min
Agilent 5977C GC/MSD
Source Temperature 280 °C
MS Quadrupole 150 °C
MS Transfer Line 280 °C
Acquisition Type SIM mode
Gain Factor 1.0
Table 1. GC and MS conditions for phthalate analysis.
Compound RT (min) Quantifier Ion (m/z) Qualifier Ions (m/z)
BB 5.133 194 105, 212
DIBP 5.395 149 150, 167
DPP 5.724 150 149, 223
DPENP 6.347 149 150, 237
BBP 7.002 149 150, 251
DBP 7.071 149 91, 150
DCHP 7.632 149 150, 167
DEHP 7.666 149 150, 167
DNOP 8.23 149 150, 249
DINP 8.554 293 127, 149
DIDP 8.898 307 149, 167
Table 2. SIM ion parameters used for data acquisition (same with helium as
carrier gas). Figure 1. System configuration. S/SL Inlet = split/splitless inlet,
PUU = purged ultimate union.
Liquid
injector
HydroInert source
witn 9 mm
extractor lens
EI source
Agilent 8890 GC
Agilent 5977C
GC/MSD
PSD
(H2
)
S/SL inlet
(H2
)
PUU
Column Restrictor
20
4
Results and discussion
Chromatographic performance
IEC 62321-8 describes a 17-minute GC method for the
determination of phthalates in polymers by GC/MS. When
using hydrogen as the carrier gas, an elution profile similar to
previous studies conducted with helium was observed. The
combination of hydrogen as the carrier gas and a smaller
diameter column resulted in a faster run time compared to
helium. The SIM chromatogram of the 1 µg/mL standard
solution containing the phthalate is shown in Figure 2. Good
chromatographic peak shapes and signal-to-noise ratio
(S/N) were obtained for 10 phthalates (excluding the internal
standard) in 15 minutes (including backflushing time).
Linearity and range
The linearity and range of the analysis were determined by
constructing solvent and standard addition calibration curves
for all phthalate compounds within the concentration range
of 0.2 to 5 mg/L. Internal standard (BB) concentration was
1 mg/L. The linearity was confirmed by observing R2
values
exceeding 0.996. Figure 3 displays representative calibration
plots for four phthalate compounds. Also, the bias percentage
for all phthalate compounds at the lowest concentration
standard fell within the acceptable range of 80 to 120% of the
actual concentration (Table 3).
Chemicals Type Origin Weight R2 %Bias STD 1
BBP Linear Include 1/x 0.99921 106.4
DIBP Linear Include 1/x 0.99849 104.9
DBP Linear Include 1/x 0.99841 111.3
DCHP Linear Include 1/x 0.99889 112.1
DEHP Linear Include 1/x 0.99902 108.0
DIDP Linear Include 1/x 0.99912 996.9
DPENP Linear Include 1/x 0.99931 104.9
DNOP Linear Include 1/x 0.99802 115.8
DINP Linear Include 1/x 0.99935 100.1
DPP Linear Include 1/x 0.99920 106.3
Table 3. Linear least-squares regression weighted calibration data for
10 phthalates.
Figure 2. SIM TIC of 10 phthalates at 1 mg/L and BB as internal standard (ISTD) at 1 mg/L.
0
0.25
0.50
0.75
1.00
1.25
1.50
1.75
2.00
2.25
2.50
2.75
3.00
Acquisition time (min)
3.5 4.0 4.5 5.0 5.5 6.0 6.5 7.0 7.5 8.0 8.5 9.0 9.5 10.0 10.5
Counts
×105
1 2 2 3 3
21
5
Relative concentratio
n
0 0.4 0.8 1.2 1.6
2 2.
4 2.
8 3.
2 3.
6
4 4.
4 4.
8 5.
2
Relative responses
0
0.2
0.4
0.6
0.8
1.0
1.2
1.4
1.6
1.8 y = 3.705611x – 0.319318 R2
= 0.9992
R = 0.9998
Relative concentratio
n
0 0.4 0.8 1.2 1.6 2.0 2.
4 2.
8 3.
2 3.
6 4.
0 4.
4 4.
8 5.
2
Relative responses
012345678
y = 1.571538x – 0.164829 R2 = 0.9984
R = 0.9997
Relative concentratio
n
0 0.4 0.8 1.2 1.6 2.0 2.
4 2.
8 3.
2 3.
6 4.
0 4.
4 4.
8 5.
2
Relative responses
0
0.1
0.2
0.3
0.4
0.5
0.6
0.7
0.8
0.9
1.0
1.1 y = 2.374077x – 0.235474 R2 = 0.9990
R = 0.9997
Relative concentratio
n
Relative responses ×1
0
1
×1
0
1
×1
0
1
BBP
DBP
DEHP
DIBP0 0.
5 1.
0 1.
5 2.
0 2.
5 3.
0 3.
5 4.
0 4.
5 5.
0
0
0.
2
0.
4
0.
6
0.
8
1.
0
1.
2
1.
4
1.
6
1.
8 y = 3.736018x – 0.332544 R2
= 0.99849070
Figure 3. Calibration curves of four phthalates in ROSH 3 from 0.2 to 5 mg/L.
22
6
Method detection limit (MDL)
The MDL was estimated based on the standard deviation (SD)
of the analysis results of nine spiked polymer samples on two
different days at 50 mg/kg. Note that phthalates were not
detected in the original polymer samples.
The MDLs for phthalate in the polymer matrix ranged
from 1.80 to 3.74 mg/kg, as illustrated in Figure 4. For all
10 phthalate compounds, the calculated relative standard
deviations (RSDs) were below 20%. These MDL values meet
the low detection limits required for phthalate analysis in
electrotechnical products.
Recovery, repeatability, and reproducibility
The recovery, repeatability, and reproducibility were
evaluated based on the results of the analysis of 14 spiked
samples on two different days. The samples were spiked at
concentrations of 50 and 500 mg/kg. The average values
of recovery obtained for the spiked samples at different
concentrations ranged from 89.6 to 101.1%, as shown in
Figure 5. Recovery between 70 and 120% is considered
satisfactory based on the limits specified in IEC 62321-8. In
addition, the %RSD of the recovery values calculated from
14 spiked samples on three days for each concentration
was less than 20%, which satisfies the requirements of
IEC 62321-8.
Figure 4. MDLs of phthalates in polymer samples.
MDL (mg/kg) RSD%
0.5
1.0
0
1.5
2.0
2.5
3.0
3.5
4.0
0%
0.5%
1.0%
1.5%
2.0%
2.5%
3.0%
3.5%
4.0%
3.65
2.15
3.37 3.57
2.71
2.19
3.36
3.07
3.74
1.80
3.52%
2.07%
3.23%
3.36%
2.60%
1.93%
2.97%
2.97%
3.27%
1.72%
BBP DIBP DBP DCHP DEHP DIDP DINP DPENP DNOP DPP
23
7
Figure 5. (A) Recovery% at 50 mg/kg of phthalate in polymer samples. (B) Recovery% at 500 mg/kg of phthalate in polymer samples.
Recovery% RSD%
Recovery% RSD%
0%
1%
2%
3%
4%
80%
85%
90%
95%
100%
105%
0%
1%
2%
3%
4%
5%
80%
85%
90%
95%
100%
105%
89.8% 90.6%
92.2%
90.2%
98.7% 98.4%
89.6%
99.3%
90.6%
3.52%
2.07%
3.23% 3.36%
2.60%
1.93%
2.97% 2.97%
3.27%
1.72%
BBP DIBP DBP DCHP DEHP DIDP DINP DPENP DNOP DPP
91.7%
89.3%
92.3%
93.0%
92.3%
96.3%
101.1%
90.3%
92.7%
89.6%
4.0%
1.1%
2.4%
2.9%
3.2%
2.2%
2.9%
2.1%
2.6%
2.8%
BBP DIBP DBP DCHP DEHP DIDP DINP DPENP DNOP DPP
90.3%
A
B
24
8
Analysis of various cable samples
All MDLs and limits of quantification (LOQs ≈ 3MDLs)
were below the lowest calibration point for all compounds.
Therefore, the reporting limits for this study were defined
as any value greater than or equal to the lowest calibration
point for the respective compound. Three real-world cable
samples were assessed for the presence of phthalate.
The chromatogram and result of three cable samples are
presented in Figure 6 and Table 4.
Compound Cable 1 Cable 2 Cable 3
DIBP Detected Detected Not detected
DPP Detected Detected Not detected
DPENP Not detected Not detected Not detected
BBP Not detected Not detected Not detected
DBP Not detected Not detected Not detected
DCHP Not detected Not detected Detected
DEHP Significant level
detected
Significant level
detected Not detected
DNOP Not detected Not detected Significant level
detected
DINP Significant level
detected Detected Not detected
DIDP Significant level
detected Detected Not detected
Not detected: below the calibration range tested
Detected: within the calibration range
Significant level detected: above the calibration range
Table 4. Phthalate result analysis of three cable samples.
Figure 6. TIC SIM of phthalates in three cable samples.
2 3
3
3
1 2 3
1 2 2 3
1 2 2 3
0
0.5
1.0
1.5
2.0
2.5
3.0
3.5
4 5 6 7 8 9 10
8.573
7.642
0
0.5
1.0
1.5
2.0
2.5
4 5 6 7 8 9 10
7.676
0
0.5
1.0
1.5
2.0
2.5
3.0
3.5
Acquisition time (min)
4 5 6 7 8 9 10
8.336
Counts Counts Counts
×107
×107
×107
25
9
Method robustness in cable matrix
When using helium gas for phthalate analysis on cable
samples, a common issue is a significant reduction on
compound responses, which cause high %RSD for certain
types of cable matrices. The method robustness when
using Agilent HydroInert source with hydrogen carrier gas,
in combination with the backflush technique, was assessed
through continuous analysis of standards and three different
cable sample matrices. The %RSD values for the response of
1 mg/L standard points of phthalates analyzed throughout
the analysis of the three cable sample matrices are presented
in Table 5.
Sample
N (Standard
Injection)
RSD Area
DIBP DPP DPENP BBP DBP DCHP DEHP DNOP DINP DIDP
Cable 1
(80 injections) 24.000 7.00 6.62 5.74 5.68 6.35 5.85 5.68 5.96 5.67 6.35
Cable 2
(30 injections) 24.000 6.11 6.16 6.08 5.83 5.72 6.30 5.96 5.66 2.41 3.44
Cable 3
(30 injections) 15.000 4.16 3.75 3.73 4.11 3.89 3.98 3.64 3.95 1.96 2.08
Table 5. The %RSD values for the response at 1 mg/L in the method robustness test.
The %RSD of the response, calculated from the 1 mg/L
standard without any maintenance (inlet, ion source),
remained below 7% for all compounds across the three cable
sample matrices. Also, the %RSD was consistently below
20% for all compounds, showcasing outstanding quantitation
stability even when continuously subjected to a complex
extract (Figure 7).
26
www.agilent.com
DE27764049
This information is subject to change without notice.
© Agilent Technologies, Inc. 2024
Printed in the USA, March 18, 2024
5994-7215EN
Conclusion
This application note demonstrates the configuration of the
Agilent 8890 GC coupled with the Agilent 5977C, using an
Agilent HydroInert source with hydrogen as the carrier gas
in combination with the backflush technique. In addition to
fulfilling the requirements of the International Electrotechnical
Commission 62321 standard for the analysis of phthalates in
electrical products, it also highlights the method's robustness
and addresses the issue of instability when analyzing
samples with complex components. This is achieved through
the self-cleaning properties of hydrogen gas within the ion
source and the use of the backflush technique to remove
components that are difficult to evaporate.
References
1. European Union (EU) Commission Delegated Directive
2015/863 of 31 March 2015 Amending Annex II to
Directive 2011/65/EU of the European Parliament and of
the Council Concerning the List of Restricted Substances,
C/2015/2067, OJ L 137, 4.6.2015, p. 10–12, http://data.
europa.eu/eli/dir_del/2015/863/oj
2. European Union Regulation No. 1907/2006. Registration,
Evaluation, Authorisation, and Restriction of Chemicals
(REACH), Establishing a European Chemicals Agency.
Annex XIV. https://eur-lex.europa.eu/legal-content/EN/TX
T/?uri=CELEX:02006R1907-20231201
3. International Electrotechnical Commission (IEC) 62321-8,
Determination of Certain Substances in Electrotechnical
Products—Part 8: Phthalates in Polymers by Gas
Chromatography/Mass Spectrometry (GC-MS), Gas
Chromatography/Mass Spectrometry Using a Pyrolyzer/
Thermal Desorption Accessory (Py/TD-GC-MS) Int.
Electrotech. Commission 2017, pp. 1–70.
Figure 7. The response values at 1 mg/L in the method robustness test.
0
1
2
3
4
5
6
7
2468 10 12 14 16 18 20 22
Cable 2 ×104
DIDP
DIDP
DIDP
DINP
DINP
DINP
DNOP
DNOP
DNOP
DEHP
DEHP
DEHP
DCHP
DCHP
DCHP
DBP
DBP
BBP
BBP
BBP
DPENP
DPENP
DPENP
DPP
DPP
DBP
DPP
DIPB
DIPB
DIPB
BB
BB
BB
0
2
4
6
8
10
12
14
246 8 10 12 14 16 18 20 22 24
Cable 1 ×105
0
2
4
6
8
10
12
0246 8 10 12 14 16
Cable 3 ×104
Application Note
Environmental
Authors
Gwen Lim Sin Yee,
CTC Analytics AG,
Zwingen, Switzerland
Aimei Zou,
Agilent Technologies, Inc.
Abstract
Growing demand for SVOC analysis at laboratories reveals the analysis bottlenecks,
e.g., a lack of practical sample preparation experience, low sample throughput, and
high consumption of chemical solvents. Online automated sample preparation
is gaining attention as a solution to address these laboratory challenges. An
automated workflow solution for quantitation of SVOCs in water samples,
combining calibration, sample preparation, and detection was developed on Agilent
gas chromatography/triple quadrupole mass spectrometer (GC/TQ) using the PAL3
robotic tool change (RTC) system in this study.
Automated Sample Preparation
Using the PAL3 RTC System for EPA
8270E Semivolatile Organic Analysis
by GC/TQ
28 Return to Table of Contents
2
Introduction
Semivolatile organic compounds (SVOCs) analysis is
widely implemented at analytical laboratories. The SW846 Compendium EPA 8270 method provides guidelines
on conditions and quality control (QC) checks to ensure
successful analysis of SVOCs using gas chromatography/
mass spectrometer (GC/MS). Furthermore, the EPA 8270E
method, which was released in 2018, includes GC/MS with an
MS/MS detector. The MS/MS selectivity ensures better lower
limit of quantitation (LLOQ) thus delivers reliable analysis
results1
.
Growing demand for SVOC analysis at laboratories reveals
the analysis bottlenecks, e.g., a lack of practical sample
preparation experience, low sample throughput, and high
consumption of chemical solvents. Online automated sample
preparation is gaining attention as a solution to address these
laboratory challenges. This application note presents a proofof-concept on a novel automated sample preparation workflow
modified based on EPA 3510C method² (that is a procedure
for isolating organic compounds from aqueous samples),
followed by Agilent gas chromatography/triple quadrupole
mass spectrometry (GC/TQ) analysis according to EPA 8270E.
The novel sample preparation workflow involved liquid-liquid
extraction (LLE) of water samples using dichloromethane
(DCM) at two specific pH conditions. The combination of these
two extracts was then analyzed by the GC/TQ. Surface water
samples were prepared automatically and online by the
PAL3 robotic tool change (RTC) system prior to GC/TQ
analysis. Likewise, working calibration standards, method
blank samples, and matrix-spiked QC samples were also
prepared automatically by PAL3. Then, 100 analytes of SOVCs
were tested and evaluated in this study.
Experimental
Instrumentation
An Agilent 7000 series triple quadrupole mass spectrometer
was coupled to an Agilent 8890 GC with a back split/splitless
inlet (SSL) and splitless inlet liner (part number 5190-2293,
900 μL, single taper, wool, Ultra). The ion source was
equipped with a 9 mm diameter drawout lens (part number
G3870-20449). The system was autotuned using the etune
algorithm embedded in the Agilent MassHunter software
version 10.1. Table 1 contains the conditions and operating
parameters for both GC and MS.
A PAL3 Series II RTC system (Figure 1) was used as a liquid
handling platform for the calibration/sample preparation
and injection onto the GC/TQ system in the study. The PAL3
system was equipped with a vortex mixer and various tray
holders and racks (for 2 mL, 10/20-mL vials). Various liquid
syringe tools were used fitting different volumes of PTFE
coated smart syringe. Solvent module and fast wash module
were also configured with the PAL3 system.
GC Conditions
Injection Volume 2.0 μL
Column Agilent J&W DB-UI8270D, 30 m x 250 μm x 0.25 μm
(part number 122-9732)
Inlet Temperature 250 °C
Injection Mode Pulsed splitless
Carrier Gas Helium, constant flow, 1.2 mL/min
Transfer Line Temperature 320 °C
Oven Program
40 °C hold for 0.5 minutes
25 °C/minute to 260 °C, hold for 9.3 minutes
5 °C/minute to 280 °C, hold for 13.3 minutes
25 °C/minute to 320 °C, hold for 18.9 minutes
MS Parameters
Acquisition Mode dMRM
Ion Source Temperature 320 °C
Quadrupole Temperature 150 °C
Ionization EI mode
EMV Mode Gain factor (10)
Solvent Delay 1.5 minutes
Cycles Per Second 15
Table 1. Agilent 8890 GC and 7000 series instrument parameters.
The integrated PAL3-GC/TQ system was controlled by
Agilent MassHunter Workstation GC/MS Data Acquisition
10.1, offering an easy user experience with a single software
system. The operating window is captured in Figure 2.
Figure 1. PAL3 RTC system on an Agilent 7000 series GC/TQ.
29
3
Calibration preparation by the PAL3 system
A total of 10 calibration levels were automatically prepared
by the PAL3 system for the experimental work in this study.
The automated procedure is illustrated in Figure 3. A stock
standard solution containing 100 analytes based on the EPA
8270E target list was manually prepared at the concentration
of 300 µg/mL (ppm) in DCM. Agilent semi-volatiles internal
standard (part number ISM-563-1, 2000 ppm) was manually
diluted by DCM at 40 ppm as ISTD. Both stock standard
solution and ISTD were loaded onto the predefined vial
positions according to the method parameters. As indicated
in Figure 3, the 3 intermediate standard solutions were
prepared from the stock standard solution by the PAL3
system, and then were used to prepare the 10 levels of
working calibration standards from 0.01 to 20 ppm. Lastly,
5 μL of ISTD were then spiked into each vial of calibration
standards at a final concentration of 2 ppm.
Sample preparation by the PAL3 system
Surface water was used as a sample to test out the
performance of automated sample preparation by the
PAL3 system. According to EPA 3510C, manual liquid-liquid
extraction (LLE) using a separatory funnel is defined for
aqueous samples, which involves large sample size and high
chemical/reagents consumption. Based on the LLE described
in EPA 3510C, an automated workflow was modified and
developed on the PAL3 system in this study. The automated
sample preparation workflow is shown in Figure 4.
1 g of NaCl was manually weighed into a 20 mL vial followed
by adding 15 mL of the water sample. The vial was capped
securely and placed on the sample rack (PAL R60 rack for
10/20-mL vial). The rest of the sample preparation workflow
steps were then done by the PAL3 system. The analytes from
the water sample were enriched 10-fold during the workflow.
Figure 2. The integrated PAL3-GC/TQ system operating window by MassHunter software 10.1.
PAL Parameters
Figure 3. Automated preparation for calibration standards by the PAL3 system.
Stock STD
300 ppm
Manual Preparation
Automation by PAL3
ISTD
40 ppm
Intermediate STD
1 2 3 4 5 6 7 8 9
10 11 12 13 14 15 16 17 18
19 20 21 22 23 24 25 26 27
28 29 30 31 32 33 34 35 36
37 38 39 40 41 42 43 44 45
46 47 48 49 50 51 52 53 54
Cal 1
0.01 ppm
Working Calibration
Standards
Cal 2
0.02 ppm
Cal 3
0.05 ppm
Cal 4
0.1 ppm
Cal 5
0.2 ppm
Cal 6
0.5 ppm
Cal 7
1 ppm
Cal 8
5 ppm
Cal 9
10 ppm
Cal 10
20 ppm
Figure 4. Automated sample preparation by the PAL3 system.
Weigh 1 g of NaCI into vial
Manual Preparation
Automation by PAL3 Add 15 mL of sample
Load sample vial on PAL3
Vortex mixing
Vortex mixing
Vortex mixing
Spike 5 µL of ISTD
Vortex mixing
Inject 2 µL onto GC
Add 50 µL of 6M NaOH
Spike target analyte
standard (or surrogate
standard)
Add 1.5 mL of DCM
Add 100 µL of 50% HSO
LLE
Vortex extraction
Phase separation 3 minutes
LLE
Vortex extraction
Phase separation 3 minutes
Transfer 50 µL lower layer
extract to 250 µL vial
Transfer 50 µL lower layer
extract to 250 µL vial
30
4
Online analysis sequence
As illustrated in Figure 5, a batch of online analysis sequence
includes working calibration standards, method blank
(MB), which is unspiked matrix blank, and matrix-spiked
QC samples. First, 10 points of calibration standards were
prepared by the PAL3 and subsequently analyzed via GC/TQ.
Next, MB was prepared by the PAL3 and immediately injected
into GC/TQ for quantitative analysis. In the meantime, PAL3
moved forward to the next sample preparation while GC/TQ
was continually working on the analysis of MB. As a result, the
integrated PAL3-GC/TQ system allowed sample preparation
and sample analysis to proceed in a parallel mode. Thus, the
overall lab productivity was increased through automation
and eliminating waiting time between runs.
Results and discussion
Compound identification
The acquisition method including multiple reaction monitoring
(MRM) transitions, collision energy, and retention time (RT)
used for this study was based on the existing well-developed
method from a previous application note3
. Figure 6 shows a
representative MRM chromatogram of the 100 analytes at
5 μg/mL (Cal 8) prepared by the PAL3 system. The symmetric
sharp peaks demonstrate the efficient chromatographic
separation of targets within the retention time window.
Figure 5. Online analysis sequence on the integrated PAL3-GC/TQ system.
PAL3 RTC
System
Preparing
Intermediate STD and
Calibration Standards
Preparing
Method Blank
(MB)
Preparing
Matrix-Spiked
QC-1
Preparing
Matrix-Spiked
QC-2
Preparing
Matrix-Spiked
QC-3
GC/TQ
System
Analyzing
Calibration
Standards
Analyzing
Method Blank
(MB)
Analyzing
Matrix-Spiked
QC-1
Analyzing
Matrix-Spiked
QC-2
Analyzing
Matrix-Spiked
QC-3
Analysis Timeline
Figure 6. Representative MRM chromatogram for 100 analytes at 5 µg/mL (Cal 8) and ISTDs at 2 µg/mL in DCM prepared by the PAL3 system.
x10 Chromatogram of 100 analytes at 5 µg/mL with ISTDs at 2 µg/mL in DCM
1
0.95
0.9
0.85
0.8
0.75
07
0.65
0.6
0.55
0.5
0.45
0.4
0.35
0.3
0.25
0.2
0.15
0.1
0.05
0
3 4 5 6 7 8 9 10
Counts vs. Acquisition Time (min)
11 12 13 14 15 16 17
31
5
Initial calibration performance
Initial calibration (ICAL) performance was evaluated in terms
of linearity, response factor (RF), and accuracy of calibration
standards. The results are summarized in Table 2. The overall
working range of the method for all analytes was determined
to be 0.01 to 20 μg/mL, while some data points for certain
compounds may be deleted at the low and high ends of the
calibration range to meet the method performance criteria
based on EPA 8270E. In this study, 96% of compounds
achieved R>0.995 (LR mode) with minimum 5 points and
97% of compounds met the accuracy requirement for each
calibration level. The %RSD of RF is within 20% for all analytes,
demonstrating the excellent performance done by the
integrated PAL3-GC/TQ system for automated calibration
preparation and acquisition analysis.
The ISTD was also assessed to determine if the method
sensitivity and stability was maintained throughout the whole
process. 5 μL of ISTD mixture was added to each calibration
level and matrix-spiked QC to reach the final concentration of
2 μg/mL. The absolute RT change for ISTDs was within the
regulatory recommendation of ≤ 30 secs. The response of all
ISTDs in the individual standard was obtained within 70 to 150%
of average response throughout the final calibration range,
meeting the EPA performance criteria1
.
Method sensitivity based on LLOQ
The method sensitivity was evaluated based on the LLOQ in
this work. The lowest point in the ICAL is defined as LLOQ
that met the performance criteria including linearity, RF, and
accuracy¹. The summary of the LLOQ for all analytes is listed
in Table 2. The LLOQ of 100 analytes was distributed across
0.01 to 0.5 μg/mL as shown in Figure 7. Overall, 39 out of 100
compounds obtained LLOQ ≤ 0.02 μg/mL, demonstrating the
excellent sensitivity of the method developed on the PAL3-
GC/TQ.
Method blanks
Method blanks (MBs) must be carried out through all stages
of sample preparation and analyzed for the compounds of
interest as a safeguard against lab contamination caused
from the sample, the reagents used, and the preparation
workflow. In this study, duplicate MBs were prepared by the
PAL3 system following the same method script except for
the addition of analytes/surrogates. Target concentration for
all compounds in MBs was obtained less than 50% of the
LLOQ, although positive presence was observed for certain
compounds, demonstrating that lab contamination was
controlled to the desired level.
Compound Name Quantifier Transition
Linearity
(LR Model)
RT
(min) RF RSD of RF
LLOQ
(μg/mL)
Recovery
(%)
RSD of Recovery
(n=3)
1,2,4-Trichlorobenzene 179.9 -> 109.0 0.9998 5.64 1.04 0.7% 0.01 115 4%
1,2-Dichlorobenzene 146.0 -> 111.0 0.9999 4.69 1.12 0.5% 0.01 109 5%
1,3-Dichlorobenzene 146.0 -> 111.0 0.9997 4.50 1.12 0.2% 0.01 105 5%
1,3-Dinitrobenzene 168.0 -> 75.0 0.9993 7.16 0.07 6.6% 0.2 102 12%
1,4-Dichlorobenzene 146.0 -> 111.0 0.9998 4.56 1.09 0.5% 0.01 105 8%
1,4-Dinitrobenzene 168.0 -> 75.0 0.9987 7.09 0.04 3.0% 0.2 114 4%
1-Bromo-2-nitrobenzene 156.9 -> 75.9 0.9995 6.56 0.20 0.8% 0.01 122 9%
1-Chloronaphthalene 162.0 -> 127.1 0.9988 6.88 1.45 2.7% 0.02 129 6%
1-Methylnaphthalene 142.0 -> 114.9 0.9998 6.48 1.59 0.7% 0.01 117 3%
1-Naphthylamine 143.1 -> 115.1 0.9951 7.70 0.29 4.3% 0.2 51 30%
2,2'-oxybis[1-chloropropane] 121.0 -> 77.0 0.9998 4.77 0.05 1.7% 0.02 108 16%
2,3,4,6-Tetrachlorophenol 230.0 -> 165.9 0.9980 7.72 0.08 7.5% 0.5 64 3%
2,3,5,6-Tetrachlorophenol 230.0 -> 165.9 0.9988 7.68 0.07 0.6% 0.5 60 4%
2,4,5-Trichlorophenol 195.8 -> 97.0 0.9995 6.70 0.35 10.2% 0.5 63 7%
2,4,6-Trichlorophenol 195.8 -> 97.0 0.9985 6.66 0.46 1.8% 0.05 64 6%
2,4-Dichlorophenol 162.0 -> 63.0 0.9996 5.57 0.98 2.0% 0.01 59 11%
2,4-Dimethylphenol 107.1 -> 77.1 1.0000 5.36 0.99 1.6% 0.01 67 14%
2,4-Dinitrophenol 184.0 -> 79.0 0.9931 7.50 0.01 3.6% 0.5 53 2%
2,4-Dinitrotoluene 165.0 -> 63.0 0.9998 7.59 0.09 3.8% 0.5 100 9%
2,6-Dinitrotoluene 165.0 -> 63.0 0.9990 7.19 0.11 1.4% 0.2 121 9%
Table 2. Analytical performance summary for analytes.
32
6
Compound Name Quantifier Transition
Linearity
(LR Model)
RT
(min) RF RSD of RF
LLOQ
(μg/mL)
Recovery
(%)
RSD of Recovery
(n=3)
2-Acetylaminofluorene 222.9 -> 181.1 0.9966 11.82 0.07 6.7% 0.5 119 5%
2-Chloronaphthalene 162.0 -> 126.9 0.9992 6.86 2.48 1.8% 0.05 118 5%
2-Chlorophenol 128.0 -> 64.0 0.9998 4.37 0.35 2.0% 0.01 54 3%
2-methyl-4,6-dinitrophenol 198.0 -> 121.0 0.9949 7.99 0.03 3.6% 0.5 53 7%
2-Methylnaphthalene 142.0 -> 141.0 0.9984 6.39 2.81 0.2% 0.01 117 4%
2-Nitroaniline 138.0 -> 92.0 0.9988 6.96 0.14 1.4% 0.5 104 9%
2-Nitrophenol 138.9 -> 81.0 0.9987 5.34 0.25 1.2% 0.01 61 9%
2-Picoline 93.1 -> 66.0 0.9997 3.20 0.28 2.3% 0.01 40 5%
3-Methylcholanthrene 268.1 -> 252.1 0.9997 15.61 0.81 1.2% 0.5 101 7%
4,4'-DDD 234.8 -> 164.9 0.9986 11.04 1.46 1.5% 0.1 123 6%
4,4'-DDE 245.8 -> 176.0 0.9984 10.56 1.15 1.3% 0.1 116 6%
4,4'-DDT 234.8 -> 164.9 0.9985 11.51 0.89 1.1% 0.05 99 8%
4-Aminobiphenyl 168.1 -> 167.1 0.9986 8.68 0.21 8.6% 0.5 59 11%
4-bromophenyl phenyl ether 248.0 -> 141.0 0.9994 8.44 0.52 1.9% 0.1 112 6%
4-chloro-3-methylphenol 107.0 -> 77.0 0.9998 6.23 0.61 1.1% 0.05 55 13%
4-Chloroaniline 127.0 -> 65.0 0.9991 5.77 0.48 2% 0.02 19 14%
4-Chlorophenyl phenyl ether 141.1 -> 115.1 0.9968 7.94 0.45 0.7% 0.02 123 2%
4-Nitroaniline 138.0 -> 108.1 0.9996 7.97 0.14 6.4% 0.5 76 8%
7,12-Dimethylbenz[a]anthracene 256.1 -> 241.1 0.9998 14.45 1.51 1.5% 0.1 121 5%
Acenaphthene 152.9 -> 77.0 0.9997 7.44 0.17 0.6% 0.01 113 6%
Acenaphthylene 151.9 -> 102.0 0.9998 7.27 0.17 0.3% 0.01 114 8%
Aldrin 262.7 -> 192.6 0.9997 9.69 0.15 1.4% 0.01 105 5%
Aniline 93.0 -> 66.0 0.9999 4.27 0.68 0.9% 0.01 23 15%
Anthracene 177.9 -> 152.0 0.9958 8.94 0.93 4.5% 0.2 118 5%
Azobenzene 77.0 -> 51.0 0.9975 8.10 1.42 2.3% 0.2 119 7%
Benz[a]anthracene 228.1 -> 226.1 1.0000 12.36 1.60 1.2% 0.5 122 7%
Benzo[a]pyrene 252.1 -> 250.1 0.9998 15.04 1.79 3.5% 0.1 113 5%
Benzo[b]fluoranthene 252.1 -> 250.1 0.9997 14.46 2.20 1.4% 0.5 120 4%
Benzo[g,h,i]perylene 276.1 -> 274.1 0.9998 17.39 1.67 3.8% 0.5 112 5%
Benzo[k]fluoranthene 252.1 -> 250.1 0.9979 14.47 1.80 0.1% 0.5 114 5%
Benzyl alcohol 108.0 -> 79.0 0.9999 4.65 0.50 0.8% 0.02 59 22%
BHC-alpha 180.8 -> 144.9 0.9995 8.44 0.50 2.3% 0.02 109 7%
BHC-beta 180.8 -> 144.9 0.9994 8.65 0.38 1.7% 0.1 117 6%
BHC-delta 218.8 -> 182.8 0.9936 8.97 0.39 1.5% 0.1 106 6%
BHC-gamma 218.8 -> 182.9 0.9991 8.74 0.35 3.0% 0.1 112 8%
bis(2-Chloroethoxy)methane 93.0 -> 63.0 0.9998 5.46 1.75 1.2% 0.01 112 10%
bis(2-Chloroethyl)ether 93.1 -> 63.0 0.9999 4.31 0.86 0.5% 0.01 101 19%
Bis(2-ethylhexyl) phthalate 149.0 -> 65.0 0.9997 12.44 1.40 1.7% 0.02 124 5%
Butyl benzyl phthalate 149.0 -> 65.0 1.0000 11.38 0.91 2.4% 0.05 128 6%
Chrysene 226.1 -> 224.1 0.9985 12.38 0.73 7.8% 0.1 111 8%
Dibenz[a,h]anthracene 278.1 -> 276.1 0.9995 16.88 0.86 4.6% 0.5 104 6%
Dibenzofuran 167.9 -> 139.1 0.9959 7.61 1.53 0.2% 0.5 116 7%
Dieldrin 262.9 -> 193.0 1.0000 10.70 0.14 1.9% 0.05 113 6%
33
7
Compound Name Quantifier Transition
Linearity
(LR Model)
RT
(min) RF RSD of RF
LLOQ
(μg/mL)
Recovery
(%)
RSD of Recovery
(n=3)
Diethyl phthalate 149.0 -> 65.0 0.9997 7.83 1.03 0.5% 0.1 114 8%
Dimethyl phthalate 163.0 -> 77.0 0.9999 7.13 0.96 0.4% 0.1 105 11%
Di-n-butyl phthalate 149.0 -> 65.0 0.9923 9.46 3.15 1.5% 0.1 125 5%
Di-n-octyl phthalate 149.0 -> 65.0 0.9993 13.90 1.94 2.7% 0.1 133 5%
Diphenylamine 167.0 -> 166.2 0.9985 8.06 0.80 3.1% 0.2 115 8%
Endosulfan I 241.0 -> 206.0 0.9997 10.41 0.08 2.0% 0.1 121 8%
Endosulfan II 240.7 -> 205.9 0.9999 11.05 0.05 1.0% 0.05 115 6%
Endosulfan sulfate 271.6 -> 236.7 0.9963 11.52 0.21 1.6% 0.02 113 8%
Endrin 262.7 -> 190.5 0.9995 10.94 0.03 0.7% 0.05 94 12%
Ethyl methanesulfonate 109.0 -> 78.9 0.9999 3.91 0.26 1.7% 0.01 79 5%
Fluoranthene 200.9 -> 199.9 0.9996 10.17 0.62 2.3% 0.1 121 7%
Fluorene 166.0 -> 165.1 0.9954 7.95 1.80 0.8% 0.1 121 6%
Heptachlor 273.6 -> 238.7 0.9999 9.37 0.19 0.7% 0.02 104 5%
Heptachlor epoxide 352.7 -> 216.7 0.9996 10.03 0.03 1.8% 0.05 112 7%
Hexachlorobenzene 283.7 -> 213.8 0.9997 8.49 0.51 2.5% 0.1 110 5%
Hexachlorobutadiene 224.7 -> 189.9 0.9998 5.83 1.26 0.9% 0.01 112 1%
Hexachlorocyclopentadiene 236.7 -> 143.0 0.9987 6.53 0.12 0.5% 0.05 87 4%
Hexachloroethane 200.9 -> 165.9 1.0000 4.99 0.88 1.0% 0.01 105 3%
Isophorone 82.0 -> 54.0 1.0000 5.27 0.82 1.4% 0.01 112 11%
Methoxychlor 226.9 -> 211.9 0.9995 12.24 0.23 0.5% 0.05 103 8%
Methyl methanesulfonate 80.0 -> 64.9 0.9999 3.91 0.05 2.0% 0.01 80 5%
Naphthalene 128.1 -> 102.1 0.9998 5.72 1.37 0.6% 0.01 113 5%
N-Nitro-o-toluidine 152.0 -> 106.0 0.9993 7.96 0.10 6.9% 0.5 71 10%
N-Nitrosodiethylamine 102.0 -> 85.0 0.9999 3.70 0.07 2.8% 0.01 97 5%
N-Nitrosodi-n-butylamine 84.1 -> 56.0 0.9998 6.08 0.14 0.2% 0.02 113 12%
N-Nitrosodi-n-propylamine 113.1 -> 71.0 0.9998 4.88 0.05 2.1% 0.02 109 12%
N-Nitrosomethylethylamine 88.0 -> 42.0 0.9999 3.25 0.11 2.3% 0.01 66 5%
N-Nitrosomorpholine 116.0 -> 86.0 0.9999 4.90 0.10 2.1% 0.05 55 13%
N-Nitrosopiperidine 114.0 -> 84.1 0.9998 5.19 0.14 2.8% 0.02 106 7%
N-Nitrosopyrrolidine 100.1 -> 55.1 0.9996 4.87 0.07 0.7% 0.05 69 13%
p-Dimethylaminoazobenzene 225.1 -> 120.1 0.9995 10.82 0.26 2.2% 0.5 144 6%
Pentachloronitrobenzene 248.8 -> 213.8 0.9997 8.70 0.17 1.2% 0.1 105 6%
Phenanthrene 177.9 -> 152.0 0.9985 8.91 1.32 2.6% 0.2 119 3%
Phenol 94.0 -> 66.1 0.9996 4.21 0.50 0.5% 0.01 19 18%
Pronamide 173.0 -> 145.0 0.9976 8.73 1.07 1.2% 0.5 116 6%
Pyrene 201.1 -> 200.0 0.9997 10.45 0.84 1.2% 0.2 119 7%
Thionazin 143.0 -> 79.0 0.9997 7.91 0.13 2.8% 0.1 118 8%
1,4-Dichlorobenzene-d4 (ISTD) 149.9 -> 114.9 N.A. 4.55 N.A. N.A. N.A. N.A. N.A.
Acenaphthene-d10 (ISTD) 161.9 -> 159.9 N.A. 7.40 N.A. N.A. N.A. N.A. N.A.
Chrysene-d12 (ISTD) 240.0 -> 235.9 N.A. 12.36 N.A. N.A. N.A. N.A. N.A.
Naphthalene-d8 (ISTD) 135.9 -> 107.9 N.A. 5.70 N.A. N.A. N.A. N.A. N.A.
Perylene-d12 (ISTD) 263.9 -> 259.9 N.A. 15.04 N.A. N.A. N.A. N.A. N.A.
Phenanthrene-d10 (ISTD) 187.9 -> 160.0 N.A. 8.88 N.A. N.A. N.A. N.A. N.A.
34
8
27 (0.01 ppm)
12 (0.02 ppm)
13 (0.05 ppm)
19 (0.1 ppm)
9 (0.2 ppm)
20 (0.5 ppm)
Figure 7. LLOQ distribution of 100 compounds.
Matrix-spiked QC recovery
Three technical replicates of matrix-spiked QC (n=3, 2 μg/mL in
the final extract) were prepared by the PAL3 system in order to
evaluate the reproducibility and robustness of the automated
sample preparation. Each QC was analyzed by GC/TQ in
duplicates account for the homogeneity of the QC solution and
the repeatability of spiked recovery. The recovery values and
%RSD are summarized in Table 2. Overall, 96% of compounds
met recovery 50 to150%, and 98% of compounds obtained RSD
of recovery ≤20% as shown in Figure 8A and 8B, respectively.
The obtained results indicate that this automated protocol
developed on the PAL3-GC/TQ is suitable, offering good
reproducibility and robustness for SVOC analysis according to
EPA 8270E.
0%
5%
10%
15%
20%
25%
30%
35%
40%
0 20 40 60 80 100
RSD of Recovery/%
Target
0
10
20
30
40
50
60
70
80
90
100
110
120
130
140
150
160
0 20 40 60 80 100
Matrix spiked QC Recovery/%
Target
8A 8B
Figure 8. Matrix-spiked QC recovery (A) at 2 μg/mL in the final extract and %RSD of recovery (B).
A B
35
Conclusion
An automated workflow solution for quantitation of SVOCs
in water samples, combining calibration/sample preparation
and detection was developed on Agilent gas chromatography/
triple quadrupole mass spectrometer (GC/TQ) using the
PAL3 robotic tool change (RTC) system in this study. The
analytical performance parameters were evaluated based
on EPA 8270E, meeting acceptance criteria for more than 90
out of 100 compounds. The PAL3 system provides various
tools and modules enabling the automated preparation of
calibration standards and samples to meet diverse customer
needs, resulting in less manual work for the user. Agilent
7000 series triple quadrupole mass spectrometer coupled to
8890 GC offers excellent selectivity and sensitivity to target
analytes. This newly developed automated workflow on the
integrated PAL3-GC/TQ system offers an easy to use and
more environmentally friendly solution for users by reducing
chemicals/standards consumption as well as waste. This
automated solution will enhance lab productivity and reduce
costs significantly.
References
1. Method EPA 8270E: Semivolatile Organic Compounds by
Gas Chromatography/Mass Spectroscopy, Revision 6,
June 2018.
2. EPA Method 3510C: Separatory Funnel Liquid-Liquid
Extraction
3. A Fast Method for EPA 8270 in MRM Mode Using the 7000
Series Triple Quadrupole GC/MS, Agilent Technologies
application note, 5994-0691EN, 2019
www.agilent.com
DE14313123
This information is subject to change without notice.
© Agilent Technologies, Inc. 2024
Published in the USA, February 1, 2024
5994-7138EN
Application Note
Environmental
Authors
Vanessa Abercrombie,
Frans Biermans,
Anastasia Andrianova,
Joel Ferrer, and Ashlee Gerardi
Agilent Technologies, Inc.
Abstract
As ionization sources continue to advance, lowering limits of detection and
increasing confidence in analyte identification, column technologies can also be
used in conjunction to push the practical limits of sensitivity and data accuracy.
Increases in the detection of analyte response also result in unwanted increases
in the detection of background noise. Gas chromatography/mass spectrometry
(GC/MS) column technology that lowers interfering column bleed ions and elevated
bleed baselines, maintains peak shape for active compounds, and can withstand
aggressive thermal cycling can greatly enhance the performance and productivity of
GC/MS methods. This application note examines how column attributes like bleed,
inertness, and thermal stability can further benefit the sensitivity and accuracy of
an MS. This study illustrates achievable data parameters, like sensitivity limits at
trace levels, retention time consistencies, and data accuracy for active semivolatile
organic compounds (SVOCs), when the Agilent 7010D triple quadrupole GC/MS
(GC/TQ) system is used.
Novel Column Chemistry Raises the
Bar on Sensitivity and Data Accuracy
in the Analysis of Semivolatile
Organic Compounds
Return to Table of Contents 37
2
Introduction
Governmental regulatory authorities have established
method and performance criteria for GC/MS measurement
of SVOCs that are identified as pollutants in environmental
and industrial matrices. The United States Environmental
Protection Agency (U.S. EPA) method 8270, for example,
contains a list of over 200 compounds, some of which
can be susceptible to unwanted chemical activity in the
instrument flow path, resulting in data quality degradation. If
the performance criteria of method 8270 are not met, system
maintenance is often needed, such as liner replacement
followed by column trimming or replacement, resulting in
unplanned instrument downtime .
Monitoring of the DFTPP tuning standard, which contains
4,4'-DDT, pentachlorophenol, and benzidine, validates the
suitability of the flow path and monitors when maintenance
should be performed. The breakdown of 4,4'-DDT to 4,4'-DDE,
and 4,4'-DDD, as well as the tailing factors of benzidine and
pentachlorophenol, tests the flow path inertness, indicating
the activity of susceptible acidic and basic analytes. GC
columns contribute the largest surface area in the sample
flow path and, therefore, are a critical factor in controlling
interferences in the analytical path. Agilent Ultra Inert (UI) GC
liners, along with an inert GC column phase, can improve the
robustness of SVOCs analyses.1
Stationary phases used in GC/MS analysis of SVOCs are
typically comprised of liquid polymers with a polysiloxane
backbone. When heat is applied to the column during routine
use, the terminal end of the stationary phase polymer can
bend back and attack itself; this is called "backbiting." Ring
structures, which are thermodynamically stable, are liberated
from the stationary phase, increasing background noise
and raising the baseline; this can be problematic for low
signal-to-noise (S/N) analytes. Peak integration can become
less repeatable, lowering quantitation accuracy. In addition,
the increase in freed ring structures—which fragment in the
ion source—and analytes can cause spectral interference in
extracted mass spectra and decrease the qualitative score
of a library spectral hit. The Agilent J&W HP-5Q and DB-5Q
GC columns have an increased thermal stability at upper
temperature limits, allowing for less spectral interference,
lower levels of column bleed, and better data quality,
especially for heavier analytes that may suffer from issues
with lower S/N.2
The new Agilent high-efficiency ion source (HES) 2.0, as
seen in Figures 1 and 2, is equipped with a novel dipolar
RF lens that redirects carrier gas and low mass ions by
> 95%. The deflected ions land on adjacent lenses and are
pumped out before entering the mass analyzer, providing
reduced noise and extended instrument robustness while
maintaining sensitivity. A ramped RF amplitude versus mass
is implemented to avoid spectrum tilt. The reduction of noise
allows for a further increase of sensitivity to attogram-level
detection limits. Built-in intelligence features such as SWARM
autotune and early maintenance feedback further enhance
instrument performance and diagnostic capabilities. The
DB-5Q GC column and the HES 2.0 work in concert to
increase the robustness of difficult analyses such as that
of SVOCs.3,4
Figure 1. Front view of the Agilent HES 2.0. Figure 2. Side view of the Agilent HES 2.0.
38
3
Experimental
The Agilent 8000 Series semivolatiles standard
(part number SVM-8270-1)—a representative mixture of
semivolatile acids, bases, and neutrals—was prepared in
dichloromethane (DCM) for calibration standards to be
analyzed at 10 to 1,000 pg on column. A semivolatile internal
standard mix (part number CRM48902) was procured from
Sigma-Aldrich (Saint Louis, MO, U.S.).
The tuning standard, containing a mixture of benzidine,
pentachlorophenol, 4,4'-diphenyltrichloroethane (4,4'-DDT),
and decafluorodiphenyltrichloroethane (DFTPP) at 25 µg/mL,
was used to obtain MS calibration and tuning settings.
A composite mixture of soils extracted with DCM prepared
for method 8270 analysis, which is a representative matrix
residue that is typically encountered in the lab, was procured
from Pace Analytical (Mt. Juliet, TN, U.S.).
An Agilent 8890 GC coupled with an Agilent 5977B GC/MSD
and Inert Extractor source, as well as an 8890 GC coupled
with a 7010D triple quadrupole GC/MS (GC/TQ) system,
upgraded with the HES 2.0, were used for the analysis.
Parameter Value
Agilent 8890 GC
Inlet 300 °C, Pulsed splitless mode
Injection Volume 0.5 mL
Inlet Liner Agilent UI inlet liner, split, low pressure drop
(p/n 5190-2295)
Injection Pulse Pressure 30 psi until 0.6 min
Purge Flow to Split Vent 50 mL/min at 0.6 min
Septum Purge Flow 3 mL/min
Oven
40 °C (0.5 min), ramp 10 °C/min to 100 °C, ramp
25 °C/min to 260 °C, ramp 5 °C/min to 280 °C,
15 °C/min to 320 °C (5 min), 10 °C/min to 330 °C
(10 min), 10 °C/min to 340 °C (10 min)
Column
Carrier Gas Helium, 1.3 mL/min, constant flow
Column
– Agilent J&W DB-5Q, 30 m × 0.25 mm, 0.25 µm
(p/n 122-5532Q)
– Agilent J&W DB-5ms UI, 30 m × 0.25 mm, 0.25 µm
(p/n 122-5532UI)
– 5ms-Type column X, 30 m × 0.25 mm, 0.25 µm
– 5ms-Type column Y, 30 m × 0.25 mm, 0.25 µm
Inlet Connection Split/splitless inlet
Outlet Connection MSD
Table 1. GC parameters for the Agilent 8890 GC.
Parameter Value
Agilent 5977B GC/MSD
Source Agilent Inert Extractor source
Mode Scan (35 to 500 amu)
Solvent Delay 2.5 min
Source Temperature 300 °C
Quadrupole Temperature 175 °C
Gain 1.0
Table 2. MS parameters for the Agilent 5977B GC/MSD.
Parameter Value
Agilent 7010D GC/TQ
Source Agilent HES
Mode Dynamic multiple reaction monitoring (dMRM)/scan
Solvent Delay 2.5 min
Source Temperature 300 °C
Quadrupole Temperature 175 °C
Gain 1.0
Table 3. MS parameters for the Agilent 7010D GC/TQ.
39
4
Peak
SVM-8270-1 Quantitative Qualitative
RT Compound Precursor Ion Product Ion Dwell CE Precursor Ion Product Ion Dwell CE
1 3.043 NDMA 74 44 56.07 6 74 42 56.07 14
2 6.411 Phenol 94 66.1 14.17 15 94 65.1 14.17 20
3 6.670 Chlorophenol-2 128 64 9.22 15 128 63 9.22 30
4 6.940 1, 3-Dichlorobenzene 146 111 8.33 15 146 75 8.33 30
4.5 6.960 1, 4-Dichlorobenzene-d4 150 115 9.31 15 150 78 9.31 3
5 7.074 1, 4-Dichlorobenzene 146 111 13.21 15 146 75 13.21 30
6 7.313 1, 2-Dichlorobenzene 146 111 9.63 15 146 75 9.63 30
7 7.700 Nitrosodi-n-propylamine N- 113.1 71 7.14 10 101 70 7.14 0
8 7.450 Methylphenol-2 (Cresol o-) 108 107 7.96 15 107 77 7.96 15
9 7.500 bis(2-Chloro-1-methylethyl)ether 121 77 7.68 5 121 49 7.68 30
10 7.700 Methylphenol-4 (Cresol p-) 108 107.1 8.36 15 107 77.1 8.36 15
11 7.800 Hexachloroethane 200.9 165.9 7.19 15 118.9 83.9 7.19 35
12 7.900 Nitrobenzene 123 77 8.28 10 77 51 8.28 15
13 8.250 Isophorone 138 82 10.46 5 82 54 10.46 5
14 8.370 Nitrophenol, 2- 138.9 81 11 15 109 81 11 10
15 8.450 Dimethylphenol 2,4- (2, 4-xylenol) 122.1 107 12.24 10 107.1 77.1 12.24 15
16 8.600 bis(2-Chloroethoxy)methane 95 65 10.37 5 93 63 10.37 5
17 8.700 Dichlorophenol, 2,4- 163.9 63 9.65 30 162 63 9.65 30
18 8.800 Trichlorobenzene, 1,2,4- 179.9 145 10.32 15 179.9 109 10.32 30
18.5 8.805 Naphthalene-d8 136.1 108.1 9.59 20 136.1 84.1 9.59 25
19 8.900 Naphthalene 128.1 102.1 12.38 20 128.1 78.1 12.38 20
20 8.980 Chloroaniline,4- 127 92 13.18 15 127 65 13.18 20
21 9.070 Hexachlorobutadiene 226.9 191.9 28.71 15 224.8 189.9 28.71 1
22 9.570 Phenol 4-chloro-3-methyl- 142 107 34.24 15 107 77 34.24 15
23 9.750 Methylnaphthalene, 2- 142.1 141.1 22.34 15 141.1 115.1 22.34 15
24 9.940 Hexachlorocyclopentadiene 237 143 17.85 20 237 119 17.85 20
25 10.060 Trichlorophenol, 2,4,5- 197.9 97 16.66 25 195.9 97 16.66 25
26 10.100 Trichlorophenol, 2,4 6- 198 97 17.45 30 196 97 17.45 30
27 10.300 Chloronaphthalene, 2- 162 127.1 21.04 20 162 77 21.04 35
28 10.400 Nitroaniline, 2- 138 92 25.89 15 138 65 25.89 25
29 10.600 Dimethyl phthalate 163 92 23.89 30 163 77 23.89 20
30 10.670 Dinitrotoluene, 2,6- 165 90.1 20.02 15 165 63 20.02 25
31 10.740 Acenaphthylene 152.1 102.1 14.15 30 151.1 77 14.15 25
32 10.840 Nitroaniline, 3- 138 92 11.18 15 138 80 11.18 5
32.5 10.826 Acenaphthene-d10 164.1 162.1 10.43 15 162.1 160.1 10.43 20
33 10.910 Acenaphthene 154.1 127 10.43 40 153.1 77 10.43 45
34 10.950 Phenol, 2,4-dinitro- 184 107 12.76 25 184 79 12.76 25
35 11.010 Nitrophenol, 4- 138.9 109 12.71 5 109 81 12.71 10
36 11.120 Dibenzofuran 168.1 139.1 13.47 25 139.1 63 13.47 35
37 11.080 Dinitrotoluene, 2,4- 165 119 12.55 5 165 63 12.55 45
38 11.340 Diethyl phthalate 149 93 12.42 15 149 65 12.42 20
39 11.460 Fluorene 166.1 165.1 10.14 15 165.1 163.1 10.14 35
40 11.470 Chlorophenyl phenyl ether, 4- 204 77 10.34 30 141.1 115.1 10.34 20
Table 4. Quantitative/qualitative transitions (dMRM based) for Agilent 7010D GC/TQ acquisition parameters.
40
5
Peak
SVM-8270-1 Quantitative Qualitative
RT Compound Precursor Ion Product Ion Dwell CE Precursor Ion Product Ion Dwell CE
41 11.480 Nitroaniline, 4- 138 108.1 10.1 5 108 80 10.1 15
42 11.510 DNOC (2-methyl-4 6-dinitrophenol) 198 167.9 13.44 5 198 121 13.44 10
43 11.630 Azobenzene 105 77.1 11.37 5 77 51 11.37 15
44 11.970 4-Bromophenyl phenyl ether 250 141 22.75 20 248 141 22.75 20
45 12.020 Hexachlorobenzene 283.8 213.9 28.52 30 248.9 214 28.52 15
46 12.220 Pentachlorophenol 265.9 167 30.92 25 165 130 30.92 25
47 12.430 Phenanthrene 178.1 152.1 22.36 25 176.1 150.1 22.36 25
47.5 12.360 Phenanthrene-d10 188.3 160.2 18.93 20 188.3 158.2 18.93 35
48 12.490 Anthracene 178.1 152.1 18.72 25 178.1 151.1 18.72 30
49 12.650 Carbazole 167 139 33.75 45 167 89 33.75 60
50 13.000 Di-n-butyl phthalate 149 121 74.98 15 149 65 74.98 25
51 13.690 Fluoranthene 202.1 152.1 26.52 30 201.1 200.1 26.52 15
52 13.980 Pyrene 202.1 151 21.27 45 201.1 200 21.27 15
53 14.900 Butyl benzyl phthalate 149 65 55.86 25 91 65 55.86 15
54 15.860 Benz[a]anthracene 228.1 226.1 23.12 30 226.1 224.1 23.12 35
54.5 15.842 Chrysene-d12 240.2 236.2 16.17 3 236.1 232.1 16.17 40
55 15.930 Chrysene 226.1 224.1 23.59 40 113.1 112.1 23.59 10
56 16.000 bis(2-Ethylhexyl) phthalate 167 149 23.09 5 149 65 23.09 25
57 17.450 Di-n-octyl phthalate 149 93 27.51 20 149 65 27.51 25
58 18.050 Benzo[b]fluoranthene 252.1 250.1 18.69 35 126 113.1 18.69 10
59 18.150 Benzo[k]fluoranthene 252.1 250.1 18.56 30 126.1 113.1 18.56 10
60 18.700 Benzo[a]pyrene 252.1 250.1 21.83 35 125 124.1 21.83 10
60.5 18.754 Perylene-d12 264.2 260.1 16.16 35 260.1 256.1 16.16 40
61 20.580 Indeno[1,2,3-cd]pyrene 276.1 274.1 30.66 40 137 136 30.66 15
62 20.660 Dibenz[a,h]anthracene 278.1 276.1 24.37 35 276.1 274.1 24.37 35
63 21.080 Benzo[g,h,i]perylene 276.1 274.1 45.33 45 138 137 45.33 1
Results and discussion
Reduced bleed stabilizes GC/MS baselines
According to EPA method 8270, the GC/MS system must
meet the required performance criteria to confirm suitability
before samples can be analyzed. The system suitability
results, along with the chromatographic resolution criteria
of closely eluting structural isomer pairs, have been
described previously for an Agilent UI glass wool liner and
UI 5ms-type column.1
The chromatographic performance of
the J&W DB-5Q GC column was tested for the suitability of
method 8270 by GC/MS, and a representative chromatogram
is demonstrated in Figure 3. When comparing the J&W
DB-5Q to a conventional 5ms-type GC column, a significant
decrease in column bleed stabilized the chromatographic
baseline, which is ideal when analyzing low concentrations of
compounds at high temperatures (Figure 4).
41
6
Figure 3. A representative chromatogram of 8,270 compounds analyzed on an Agilent J&W DB-5Q GC column and collected using an Agilent 5977B GC/MSD.
Acquisition time (min)
5 6 7 8 9 10 11 12 13 14 15 16 17 18 19 20 21 22 23 24
0
20
40
60
80
100
10
30
50
70
90
Counts (%) Figure 4. A standard of 50 pg on column of 8,270 compounds, analyzed on an Agilent J&W DB-5Q (blue) and the 5ms-type column Y (red), and collected on an
Agilent 5977B GC/MSD.
17 18 19 20 21 22 23 24
1
3
5
7
9
11
2
4
6
8
10
Acquisition time (min)
Counts (%)
42
7
Reduced column activity improves peak symmetry of
problematic analytes
In EPA method 8270, column activity is observed as
increased peak tailing, resulting in S/N loss. This is commonly
observed with the more active compounds in the analyte
panel. In Figure 5, the peak symmetry and S/N ratio for
2,4-dinitrophenol, a problematic analyte, was compared at
250 pg on column, analyzed on both a DB-5Q column and
a conventional 5ms-type column. The column activity of
the 5ms-type column X resulted in a loss of peak symmetry
that significantly decreased analyte sensitivity. Also, the
peak shape of another problematic compound, 2-methyl4,6-dinitrophenol, was compared through analysis on two
conventional 5ms-type columns (marked as X and Y) and
the DB-5Q, as shown in Figure 6. The inertness of the DB-5Q
allowed for better S/N, leading to better sensitivity for this
difficult phenolic compound.
Lastly, Figure 7 demonstrates the comparison of
pentachlorophenol on the DB-5Q and a conventional 5ms-type
column. Again, when analyzed at the same concentration, the
tailing factor of pentachlorophenol increased, leading to less
S/N response. When working with difficult analytes, such as
those in EPA method 8270, inert column chemistries, such as
those observed on the DB-5Q, will optimize sensitivity.
Figure 5. Integrated peak 250 pg on column 2,4-dinitrophenol, analyzed on
the 5ms-type column X and an Agilent J&W DB-5Q GC column.
Symmetry: 2.3
S/N: 715.2
Acquisition time (min)
10.7 10.8 10.9 11.0 11.1
0
0.5
1.0
1.5
2.0
2.5
3.0
3.5 Agilent J&W DB-5Q
Acquisition time (min)
10.6 10.7 10.8 10.9 11.0
0
1
2
3
4
5
6
7
8
9
5ms-Type column X
Symmetry: 4.47
S/N: 8.83
×102
×103
Counts (%) Counts (%)
A
B
43
8
Figure 6. Integrated peak 250 pg on-column 2-methyl-4,6-dinitrophenol,
analyzed on the 5ms-type columns X and Y, and an Agilent J&W DB-5Q
GC column.
×104
Acquisition time (min)
11.3 11.4 11.5 11.6
0
0.1
0.2
0.3
0.4
0.5
0.6
0.7
0.8
0.9
1.0
1.1
1.2
1.3 Symmetry: 1.7
S/N: 310.5 Agilent J&W DB-5Q
C
Counts
×103
Acquisition time (min)
11.2 11.3 11.4 11.5 11.6
0
0.1
0.2
0.3
0.4
0.5
0.6
0.7
0.8
0.9
1.0
1.1
1.2
Symmetry: 6.9
S/N: 8.3 5ms-Type column Y
B
Counts
×103
Acquisition time (min)
11.2 11.3 11.4 11.5 11.6
0
0.5
1.0
1.5
2.0
2.5
3.0
3.5
4.0
4.5 Symmetry: 2.4
5ms-Type column X S/N: 212.9
2-Methyl-4,6-dinitrophenol A
Counts
Figure 7. Integrated peak 250 pg on-column pentachlorophenol, analyzed
the 5ms-type column Y and an Agilent J&W DB-5Q GC column.
Pentachlorophenol
Acquisition time (min)
12.0 12.1 12.2 12.3 12.4
0
0.5
1.0
1.5
2.0
2.5
3.0 Symmetry: 2.1
S/N: 245.0
Agilent J&W DB-5Q
Acquisition time (min)
11.9 12.0 12.1 12.2 12.3
0
0.2
0.4
0.6
0.8
1.0
1.2
1.4
1.6
1.8
2.0 Symmetry: 3.2
S/N: 82.8 5ms-Type column Y
×104
×104
A
Counts Counts
B
44
9
Improved durability leads to consistent chromatography
To stress the robustness of the GC system in a real-world
application, a heavy soil matrix was diluted in DCM and
analyzed over multiple thermal cycles. DFTPP tuning standard
was analyzed every five matrix injections, using %DDT
breakdown as an indicator to replace the inlet liner. The inlet
liner and septum were replaced every 20 matrix injections,
as they failed the method criteria after %DDT breakdown.
The peak shapes of pentachlorophenol and benzidine were
used as indicators of increased column activity resulting
from matrix accumulation and thermal degradation. Figure 8
demonstrates that after 200 matrix injections, the retention
times and peak shapes for all test compounds were
consistent with minimal data quality reduction. The DB-5Q
column is durable to withstand routine, high-throughput
cycling shape, even when working with difficult matrices such
as soil extracts.
Figure 8. DFTPP tune mix initially (black) and after 200 matrix injections (blue) on an Agilent J&W DB-5Q column.
Acquisition time (min)
8 9 10 11 12 13 14 15 16
1 2
3
4
Tune mix after 200 matrix injections
Tune mix initially
Peak Compound
1 Pentacholophenol
2 DFTPP
3 Benzidine
4 DDT
45
10
Optimal sensitivity and acquisition flexibility
The improved sensitivity of the HES 2.0 ion source, with the
triple off-axis detector configuration, allows for fast MRM
speeds. Data can now be acquired in dMRM and scan modes
simultaneously. This improvement allows for targeted and
untargeted analyses at the same time. The dMRM is useful
in setting up MRM methods, as once the retention time is
inputted into Agilent MassHunter acquisition software, the
dwell time is calculated automatically. While this streamlines
the method setup process, if retention times shift from matrix
accumulation or thermal instability, it can be helpful to collect
scan data and dMRM in the same acquisition method. In
Figures 9 and 10, a 10 pg on-column standard was analyzed
using dMRM/scan collection mode. Figure 9 demonstrates
the extracted scan chromatogram, which is zoomed-in on
later-eluting compounds to display their S/N ratios. With
the combination of the improved HES 2.0 ion source, along
with the improved thermal stability of the DB-5Q column, it is
possible to simultaneously acquire selective methods, such
as dMRM and scan mode.
Selectivity matching eases column adoption
A standard EPA method 8270 analysis was conducted at
1,000 pg on-column, using the same instrumentation and
method conditions, on a DB-5ms UI and a DB-5Q column. The
similar selectivity allows for upgrading analytical methods
without the need for more development, as seen in Figure 11.
Also, with the same selectivity, there is no need to update
retention times, which makes the DB-5Q compatible with
existing retention time locking libraries.
Figure 9. A standard of SVOCs analyzed at 10 pg on column, collected by dMRM/scan mode with the extracted scan data.
20.5 20.6 20.7 20.8 20.9 21.0 21.1 21.2
m/z = 276
m/z = 278
m/z = 279
m/z = 274
61
62
63
Acquisition time (min)
Acquisition time (min)
345678 9 10 11 12 13 14 15 16 17 18 19 20 21 22 23 24 25 26 27 28 29 30 31 32 33 34 35 36 37 38 39 40 41 42 43 44 45 46
10
30
50
70
90
0
20
40
60
80
10 pg On-column of SVM-8270-1, collected in scan mode
Counts (%)
Peak Compound S/N
61 Indeno(1,2,3-cd)pyrene 14.53
62 Dibenz[a,h]anthracene 11.03
63 Benzo[g,h,i]perylene 25.72
46
11
Figure 11. The Agilent J&W DB-5Q has similar selectivity to an Agilent J&W DB-5ms UI, as demonstrated in the analysis of 8,270 compounds.
Agilent DB-5ms UI
Agilent DB-5Q
Figure 10. A standard of SVOCs analyzed at 10 pg on column, collected by dMRM/scan mode with the extracted MRMs.
Acquisition time (min)
5 6 7 8 9 10 11 12 13 14 15 16 17 18 19 20 21
0
10
20
30
40
50
60
70
80
90
100
Counts (%)
61
62
63
Acquisition time (min)
20.3 20.4 20.5 20.6 20.7 20.8 20.9 21.0 21.1 21.2
Peak Compound S/N Ratio
61 Indeno(1,2,3-cd)pyrene 157.8
62 Dibenz[a,h]anthracene 81.9
63 Benzo[g,h,i]perylene 208.5
47
www.agilent.com
DE-000135
This information is subject to change without notice.
© Agilent Technologies, Inc. 2024
Printed in the USA, August 14, 2024
5994-7686EN
Conclusion
This application note demonstrates that the Agilent J&W
DB-5Q GC column can exceed the performance requirements
of EPA method 8270. Agilent Ultra Inert chemistry across the
sample flow path will maintain peak symmetry of problematic
analytes, leading to improved limits of detection and accurate
integration. Ultralow-bleed chemistry stabilizes baselines
and reduces interfering bleed ions. High-temperature
stability allows for the repeated temperature cycling needed
for high throughput methods, even when analyzing heavy,
complex soil matrices. The matching selectivity of the J&W
DB-5Q compared to the Agilent J&W DB-5ms UI allows for
seamless adoption, including compatibility with existing
retention time locking libraries. The analytical performance
of the DB-5Q coupled with the upgraded Agilent HES 2.0
allows for optimal sensitivity, as well as the ability to perform
targeted and nontargeted analyses in tandem.
References
1. Smith Henry, A. Comparison of Fritted and Wool Liners
for Analysis of Semivolatile Organic Compounds by
Gas Chromatography/Mass Spectrometry, Agilent
Technologies application note, publication number
5994-2179EN, 2020.
2. How Does Bleed Impact GC/MS Data and How Can It
Be Controlled? Agilent Technologies technical overview,
publication number 5994-7586EN, 2024.
3. Andrianova, A.; Zhao, L. Brewing Excellence: Quantitating
Over 200 Pesticides in Black Tea with Steady
Performance and Maximized Uptime by GC/MS/MS,
Agilent Technologies application note, publication number
5994-7436EN, 2024.
4. Reaser, B. C. Enhanced Longevity and Revolutionized
Robustness for the Sensitive Detection of 190 Pesticides
over 800 Injections, Agilent Technologies application note,
publication number 5994-7385EN, 2024.
Application Note
Environmental
Authors
Luann Wong, Gabrielle Black,
and Thomas Young
Department of Civil and
Environmental Engineering,
University of California, Davis,
CA, U.S.
Sofia Nieto
Agilent Technologies, Inc.
Abstract
Development of accurate mass libraries in environmental applications is key in
expanding the scope of monitored compounds and allowing for target/suspect
detection with high confidence. It also provides the opportunity to use a targeted
data analysis approach that offers higher sensitivity and flexibility compared to
nontarget screening.
This application note describes the development and use of an accurate mass
personal compound database and library (PCDL) of per- and polyfluoroalkyl
substances (PFAS) for the Agilent 7250 GC/Q-TOF and demonstrates how the
PCDL can be applied in both target as well as nontarget screening approaches
using environmental samples, such as drinking water extracts. This study also
demonstrates the benefits of using the high-resolution accurate mass GC/Q-TOF
in nontarget screening using NIST23 and third-party libraries for identifying a
substantial number of other contaminants of industrial origin in drinking water.
Accurate Mass Library for PFAS
Analysis in Environmental Samples
and Workflow for Identification of
Pollutants in Drinking Water Using
GC/Q-TOF
Return to Table of Contents 49
2
Introduction
PFAS are emerging contaminants of increasing concern due
to their environmental persistence, toxicity, and capability
of bioaccumulation. There are currently thought to be over
6,000 PFAS that have been commercially produced1
, and
recent studies have shown that many emerging PFAS
detected in the environment can be volatile or semivolatile
in nature2–4. Therefore, many analytical techniques are
necessary for PFAS detection. Gas chromatography/mass
spectrometry (GC/MS) is typically used for detecting
volatile and semivolatile nonpolar PFAS compounds. In
this study, the 7250 GC/Q-TOF system was used to take
advantage of its high-resolution for detecting compounds
with mass defects that are different from that of complex
environmental matrixes.
To ensure the most sensitive and reliable detection of PFAS,
an accurate mass library that includes over 150 electron
ionization (EI) PFAS spectra and contains both retention times
(RTs) and retention indices (RIs) was created.
The PFAS PCDL was further tested using both target and
nontarget approaches when analyzing the drinking water
extracts. In addition, to fully benefit from the GC/Q-TOF
accurate mass capability combined with full-spectrum
acquisition, enabling nontarget detection, NIST23 and
the third-party library MassBank of North America
(MassBank.us5
) were also used to identify other contaminants
in drinking water, with the false positives being effectively
removed based on accurate mass information. Thus,
many pollutants were identified in drinking water, including
disinfection by-products (DBPs), industrial chemicals
originated from personal care products, pharmaceuticals, as
well as pesticide residues.
Experimental
Sample preparation
The drinking water samples were collected at two different
locations in California, U.S. and represented two different
water source categories: a small surface water (Weaverville)
and a mixed surface and ground water (Irvine). Water
samples (2.4 L) were extracted on a multimode solid phase
extraction (SPE) using HLB, WAX, WCS, and Isoelut ENV
sorbents, and eluted with 5% methyl tert-butyl ether (MTBE)
in methanol (MeOH), dichloromethane (DCM), 0.5% NH4
OH
in 1:1 ethyl acetate (EtAc):MeOH, and 1.7% formic acid in
1:1 EtAc:MeOH. The combined extracts were concentrated,
solvent exchanged to EtAc, and diluted tenfold.
Table 1. Data acquisition parameters.
GC and MS Conditions Agilent DB-5ms Agilent DB-624
MS Agilent 7250 GC/Q-TOF
GC Agilent 8890 GC
Inlet Agilent multimode inlet, Ultra Inert 4 mm liner, single taper
with wool
Inlet Temperature 70 °C for 0.01 min; 300 °C/min to 250 °C
Injection Volume 1 µL
Column
Agilent J&W DB-5ms Ultra
Inert (UI), 30 m × 0.25 mm,
0.25 µm
Agilent DB-624 Ultra Inert,
30 m × 0.25 mm, 1.4 µm
Oven Temperature
Program
35 °C for 2 min;
7 °C/min to 210 °C,
20 °C/min to 300 °C,
4 min hold
30 °C for 2 min;
3 °C/min to 75 °C,
2 °C/min to 110 °C,
10 °C/min to 210 °C,
20 °C/min to 240 °C,
2 min hold
Column Flow 1.2 mL/min constant flow 1 mL/min constant flow
Carrier Gas Helium
Transfer Line
Temperature
250 °C
Quadrupole
Temperature
150 °C
Source Temperature 200 °C
Electron Energy 70 eV
Emission Current Variable by time segment, 0.01 to 5 µA
Spectral Acquisition
Rate
5 Hz
Mass Range (Tune) 50 to 1,200 m/z
Data acquisition and data processing
GC/MS analysis was performed using an Agilent 8890
GC coupled to an Agilent 7250 GC/Q-TOF using the data
acquisition parameters described in Table 1. PFAS accurate
mass spectra of GC-amenable compounds were acquired
from individual PFAS standards.
The chromatographic deconvolution and library search
were performed in Agilent MassHunter Unknowns Analysis
software, version 11.1. Accurate mass electron ionization (EI)
fragments were converted to the theoretical m/z using Agilent
MassHunter Qualitative Analysis software, version 10.0, and
the spectra were exported into the accurate mass Agilent
Personal Compound Database and Library (PCDL) Manager,
version 8.0. The Agilent GC/Q-TOF Pesticide PCDL, PFAS
PCDL, NIST23, as well as MassBank.us were used to perform
initial compound identification. Prior to performing the library
search with MassBank.us, the spectra, along with metadata
information from this database, were exported in the PCDL
format using Agilent ChemVista software, version 1.0, as
described elsewhere.6–7 RIs and accurate mass information
were used to confirm the compound identification. Statistical
analysis was performed in Agilent Mass Profiler Professional
(MPP), version 15.1.
50
3
Results and discussion
Accurate mass library for PFAS
To create an accurate mass GC/MS PCDL, spectra
were collected for over 100 volatile and semivolatile
PFAS compounds. Accurate mass fragment ions were
automatically annotated with formulas based on accurate
mass information and isotope ratios using MassHunter
Qualitative Analysis software (Figure 1). The fragment
formula annotations were verified, corrected when necessary,
and automatically converted to the theoretical m/z.
Figure 1. (A) Extracted ion chromatogram (EIC) of the molecular ion and fragment formula annotation of spectrum for one of the PFAS compounds in Agilent
MassHunter Qualitative Analysis software. (B) The PFAS PCDL contains EI spectra as well as the metadata, including molecular structure and database identifiers.
0
1
2
3
19.718
17.0 17.5 18.0 18.5 19.0 19.5 20.0 20.5 21.0 21.5 22.0 22.5 23.0
0
0.2
0.4
0.6
0.8
1.0
1.2
1.4
1.6 107.0303
[C4 H5 F2 O]+
68.9947
[C F3]+
130.9914
[C3 F5]+
376.0138
168.9879 [C9 H5 F13 O]+
[C3 F7]+
245.0003
[C6 H2 F9]+
195.0034
[C5 H2 F7]+
289.0065
[C8 H3 F10]+
337.0078
[C9 H4 F11 O]+
60 80 100 120 140 160 180 200 220 240 260 280 300 320 340 360 380
3-(Perfluorohexyl)-1,2-epoxypropane
×106 A
B
Acquisition time (min)
Counts
×106
Counts
Mass-to-charge (m/z)
51
4
The PFAS compound classes include perfluoroalkyl iodides
(PFAIs), fluorotelomer iodides (FTIs), fluorotelomer alcohols
(FTOHs), fluorotelomer olefins (FTOs), fluorotelomer
acrylates (FTACs), fluorotelomer methacrylates (FTMACs),
fluorotelomer carboxylic acids (FTCA), fluorotelomer
unsaturated carboxylic acids (FTUCA), perfluoroalkane
sulfonamides (FASA), and more (Figure 2).
To acquire spectra for the PFAS PCDL, the mid-polar DB-624
GC column (30 m × 0.25 mm, 1.4 µm) was used to ensure the
best retention and separation of the challenging volatile PFAS.
In addition to the RTs, RIs for the mid-polar column phase
were also calculated for all compounds. Inclusion of RIs
provides the flexibility of the GC method when using the PFAS
PCDL as soon as GC column phase stays the same.
The remaining metadata, including compound structures and
database identifiers, were added using PCDL Manager.
Compound overlap of the volatile and semivolatile PFAS
classes between the accurate mass PFAS PCDL and NIST23
library is shown in Table 2.
Table 2. Compound overlap between the PFAS PCDL and NIST23 library.
Percent Unique to PCDL Total Number
All 53 158
PFCA 55 29
FTO 50 6
PFAI and FTI 17 6
FTCA and FTUCA 67 9
FTAC and FTMAC 25 8
FTOH 40 15
FASA 8 12
A significant number of the PFAS compounds (over 50%) were
found to be present uniquely in the PFAS PCDL. In particular,
the spectra of many per- and polyfluorinated carboxylic acids,
fluorotelomer olefins, and fluorotelomer alcohols were found
to be unique to the PCDL, thus highlighting the value of the
accurate mass PFAS library in PFAS research.
Figure 2. Examples of different PFAS compound classes from the PFAS PCDL.
+EI MS1 QTOF FV=70
60 80 100 120 140 160 180 200 220 240 260 280 300 320 0
20
40
60
80
100
+EI MS1 QTOF FV=70
40 60 80 100 120 140 160 180 200 220 240 260 280 300 320 340 360 0
20
40
60
80
100
+EI MS1 QTOF FV=70
30 50 70 90 110 130 150 170 190 210 230 250 270 290 0
20
40
60
80
100
+EI MS1 QTOF FV=70
40 80 120 160 200 240 280 320 360 400 440 480 520 0
20
40
60
80
100 55.01784
100.00
99.04405
37.80 168.98828 21.40
3.92 426.99982
1.41 1.30 3.15
230.98508
1.04
+EI MS1 QTOF FV=70
60 80 100 120 140 160 180 200 220 240 260 280 300 320 340 360 380 400
Abundance
0
20
40
60
80
100
+EI MS1 QTOF FV=70
40 60 80 100 120 140 160 180 200 220 240 260 280 300 320 340 360 380
Abundance
0
20
40
60
80
100
+EI MS1 QTOF FV=70
60 80 100 120 140 160 180 200 220 240 260 280 300 320 340 360 380
Abundance
0
20
40
60
80
100
+EI MS1 QTOF FV=70
60 80 100 120 140 160 180 200 220 240 260 280 300 320 340 360
Abundance
Abundance Abundance Abundance Abundance
0
20
40
60
80
100
218.98508
100.00
68.99466
59.25 126.90392
32.40 29.32 176.90073
20.56 99.99306
11.20 3.55
Nonafluoro-1-iodobutane (PFBI)
1,1,1,2,2,3,3,4,4-Nonafluoro-6-iodohexane (6:2 FTI)
8:2 Fluorotelomer acrylate (8:2 FTAC)
Perfluoroheptanoic acid (PFHpA)
Methyl perfluorohexanoate
6:2 Fluorotelomer alcohol (6:2 FTOH)
Perfluorooct-1-ene
Mass-to-charge (m/z)
Mass-to-charge (m/z)
Mass-to-charge (m/z)
Mass-to-charge (m/z)
Mass-to-charge (m/z)
Mass-to-charge (m/z)
Mass-to-charge (m/z)
Mass-to-charge (m/z)
238.89754
345.88956
312.98813 380.97549
518.01691
68.99466
100.00 130.99147
50.23 99.99306
21.27
180.98828
16.76
281.00186 15.55 218.98508
6.61 246.98000
1.85
308.99680
1.51
146.98639
1.08
68.99466
100.00 118.99147 45.56 318.97870
23.32
168.98828
22.49 230.98508 51.00408 21.05 2.30 280.98187
1.02
69.03349
100.00
268.03287
89.62
95.01031
8.74
51.00408
8.37
118.99147 8.33 253.00940
5.26 204.03682
1.48 149.04086
1.45
380.97549 68.99466 100.00 84.54 130.99147
74.31
180.98828
31.56 92.99466
22.98 230.98508
11.52 280.98187
5.32
330.97870
4.05
95.01031
100.00 127.01654 48.66 296.00537 51.00408 21.59 10.86 168.98828
6.72
230.98508
5.98
363.00491
5.70
373.92084
100.00
227.01015
40.57 140.91957
30.78
68.99466
29.23 247.01639
0.74 354.92245
0.06
285.92725
0.05
2,2,3,3,4,4,4-Heptafluorobutyl methacrylate (3:1 FTMAC)
52
5
PFAS in drinking water extracts
For PFAS detection, the extracts of drinking water were
separated on a DB-624 column and analyzed using the
7250 GC/Q-TOF. To be able to detect early-eluting volatile
PFAS, the emission current was set up by segment, as shown
in Table 1, thus excluding the solvent peak from the detection.
Both target and nontarget approaches were evaluated using
the PFAS PCDL. When performing the nontarget analysis,
the chromatographic deconvolution was carried out in the
MassHunter Unknowns Analysis software using a SureMass
algorithm, which is optimized for complex, high-resolution
EI data. The PFAS PCDL then was used to search the
deconvoluted spectra with RT matching. One of the PFAS—a
transformation product of the perfluorocarboxylic acid—
was identified in drinking water extract using this approach
(Figure 3A). An additional benefit of nontarget screening is
the use of multiple libraries (including libraries containing unit
mass spectra) that can all be searched simultaneously.
Figure 3. Example of PFAS (methyl perfluorooctanoate) identified in drinking water samples using PFAS PCDL in a nontarget approach using (A) Agilent
MassHunter Unknowns Analysis software, and (B) a target GC/Q-TOF screening approach.
A
B
53
6
One of the advantages of the target approach based on the
GC/Q-TOF Screener tool of the MassHunter Quantitative
Analysis software (described in detail previously8
) and PCDL
is that all the parameters could be set up individually for
every compound in the method. This approach allows for a
substantial flexibility when performing the screening method
optimization at the data processing level, enabling the highest
degree of sensitivity and specificity. Another significant
benefit of this approach is that it saves the time usually spent
reviewing the results. The GC/Q-TOF Screener algorithm
validates quantifier and qualifier ions based on outliers, and
for most compounds, either confirms or rejects their presence
automatically. Only a few compounds remain highlighted
to indicate that a manual review might be necessary for
confirmation of compound identity.
The same PFAS compound—methyl perfluorooctanoate—that
was identified in drinking water extracts using a nontarget
approach was also detected using the GC/Q-TOF Screener
with library match scores (LMS) of > 99 (Figure 3B). It has
previously been reported that perfluoroalkyl carboxylic acids
can be converted to corresponding methyl esters in the
presence of methanol9
, thus plausibly explaining the presence
of the methyl ester of PFOA in drinking water extracts.
Identification of other contaminants in drinking
water samples
To screen for additional contaminants in drinking water
samples in a nontarget manner, the GC/Q-TOF Pesticide
PCDL, NIST23 library, and MassBank.us were used. The
choice of the DB-5ms UI column enabled RI matching
while searching the NIST23 library, thus enhancing the
confidence in compound identification. The ExactMass
tool in MassHunter Unknowns Analysis software was
used to eliminate the false positives based on the accurate
mass information and molecular formula of the hit. This is
particularly practical when using unit mass libraries such as
NIST23 (Figure 4A) and MassBank.us.
Over 100 contaminants were identified and confirmed using
accurate mass information (Figure 4A and 4B, and Tables 3
and 4) from the sample without re-injection.
Among the identified contaminants, one of the significant
groups was disinfection by-products, formed when
chlorine and bromine interact with organic matter. These
compounds included halomethanes and haloacetic acids,
which are the most common disinfection by-products. Other
prominent groups of contaminants included compounds
originating from industrial processes (such as those used
in cleaning products and manufacturing of plastics, dyes,
and pharmaceuticals), PAHs and their derivatives, as well
as pesticides.
Approximately 400 hits per sample with LMS > 70
were detected using MassBank.us, including over
20 contaminants—mostly DBPs and PAHs. Since this library
does not contain RI information for most of the compounds,
many hits could potentially be false positives. One such
example is shown in Figure 5. The hit from MassBank.us was
1-bromooctane with a high LMS of 88.3 and an RI of 1,134,
according to NIST23. This would make a difference of over
400 RI units between the hit and the compound in question,
indicating that the ID is likely incorrect. The difference
between the compound RI and the NIST23 hit was only 10 RI
units (Figure 5B). Note that the hits from both NIST23 and
MassBank.us perfectly match the accurate mass information
displayed in the ExactMass tables (Figure 5).
54
7
Figure 4. Examples of the contaminants identified in drinking water extracts using (A) NIST23 and (B) Agilent GC/Q-TOF Pesticide PCDL. The ExactMass tool
(outlined in orange) helped to provide additional confirmation of unit mass library hits based on accurate mass. Compound ions are highlighted in the mirror plot
when m/z corresponds to the library hit formula.
A
B
55
8
Table 3. Contaminants identified in drinking water using the NIST23 library with LMS > 75. *The cases where delta RI was calculated considering predicted RIs
rather than experimental (experimental not available) are denoted by an asterisk. Some of the prominent disinfection by-products are highlighted in red.
RT Compound Name
Match
Score Formula
RI
Difference
4.79 Bromodichloromethane 95.4 CHBrCl2 –56
4.81 Chloral 78.8 C2
HCl3
O –9
4.91 Dichloroacetonitrile 86.4 C2
HCl2
N –76
4.95 Chloromethylmethyl sulfide 94 C2
H5
ClS –59*
5.11 Dimethyl disulfide 98.4 C2
H6
S2 –35
5.35 Methyldiallylamine 85.7 C7
H13N –50*
5.47 Bromoacetonitrile 82.8 C2
H2
BrN 1
5.95 Dibromochloromethane 95.5 CHBr2
Cl –25
6.01 Tetrachloroethylene 96.5 C2
Cl4 –12
6.04 1,1-Dimethyl-3-chloropropanol 88.4 C5
H11ClO 7
6.34 Bromoacetone 87.8 C2
HBrClN –3
6.59 Dichloroacetic acid methyl ester 89.2 C3
H4
Cl2
O2 –7*
7.67 Tribromomethane 98.2 CHBr3 –10
8.24 Methyl bromo(chloro)acetate 77.4 C3
H4
BrClO2 –3
8.31 Dibromoacetonitrile 86.6 C2
HBr2
N –15
10.63 2,2-Dichloroacetamide 83.5 C2
H3
Cl2
NO –4*
10.73 1,2-Dichlorbenzene 98.5 C6
H4
Cl2 9
14.12 Naphthalene 81.9 C10H8 –4
15.45 Caprolactam 89.6 C6
H11NO 3
16.43 2-Methylnaphthalene 89.3 C11H10 –1
16.64 Phthalic anhydride 92.5 C8
H4
O3 5
16.98 Benzamide 82.8 C7
H7
NO 18
18.05 Biphenyl 83.2 C12H10 –1
18.18 Benzeneacetamide 84.3 C8
H9
NO 13
19.27 Dimethyl phthalate 75.1 C10H10O4 8
19.96 Acenaphthene 91.2 C12H10 –4
20.18 4-Methylbiphenyl 79.4 C13H12 –4
20.28 2,4-Di-tert-butylphenol 90.2 C14H22O 10
20.56 Dibenzofuran 92.4 C12H8
O –5
21.23 1-Bromododecane 75.7 C12H25Br –10
21.45 Diethyltoluamide (DEET) 78.1 C12H17NO 10
21.69 Diethyl phthalate 96 C12H14O4 8
21.71 Fluorene 75.3 C13H10 –4
22.01 2-(Methylmercapto)benzothiazole 77.8 C8
H7
NS2 2
22.43 Benzophenone 94.8 C13H10O 4
22.60 Tributyl phosphate 93 C12H27O4
P 7
23.51 Hexachlorobenzene 97.2 C6
Cl6 9
RT Compound Name
Match
Score Formula
RI
Difference
24.19 9H-Fluoren-9-one 97.1 C13H8
O 8
24.26 9H-Fluoren-9-ol 81.5 C13H10O 9*
24.90 Anthracene 94.4 C14H10 0
24.91 Tris(2-chloroisopropyl)phosphate 82.2 C9
H18Cl3
O4
P 27
25.02 Benzo[h]quinoline 88.2 C13H9
N –2
25.53 2,4-Diphenyl-4-methyl-2(E)-pentene 76.5 C18H20 8
25.55 Benzo[f]quinoline 91.1 C13H9
N –1
25.73 Carbazole 76.8 C12H9
N –4
25.96 Di-sec-butyl phthalate 90.8 C16H22O4 –2
26.09 3,3-Diphenyl-2-propenenitrile 82.7 C15H11N 18*
26.27 3-Methyldibenzothiophene 80.8 C13H10S –5
26.66 3-Methylphenanthrene 84 C15H12 1
27.00 2-Methylanthracene 88.5 C15H12 –27
27.31 Dibutyl phthalate 92.4 C16H22O4 9
27.59 9,10-Anthracenedione 93.2 C14H8
O2 –27
28.21 Octachlorostyrene 88.6 C8
Cl8 –7
28.36 Cyclic octaatomic sulfur 93.1 S8 –18
28.51 Drometrizole 82.3 C13H11N3
O –5
28.53 Fluoranthene 97.8 C16H10 –12
28.54 Phenindione 79 C16H10 –28*
28.91 Dibenzothiophene sulfoxide 87.2 C12H8
OS –41*
28.94 Pyrene 89.3 C16H10 –25
29.02 1-Azapyrene 78.4 C15H9
N 2
29.34 Bisphenol A 84.1 C15H16O2 26*
29.37 2-Amino-9-fluorenone 83.3 C13H9
NO 2*
29.69 Bis(4-chlorophenyl) sulfone 77 C12H8
Cl2
O2
S –1
29.87 2,2'-Methylene-bis-(4-methyl-6-tbutylphenol) 87.5 C23H32O2 2
30.79 Benzo[b]naphtho[1,2-d]thiophene 77.2 C16H10S 13
30.90 7H-Benz[de]anthracen-7-one 89.2 C17H10O 85
30.94 Benzo[b]naphtho[2,1-d]thiophene 81.3 C16H10S –16
31.04 Phthalic acid, di(2-propylpentyl)
ester 94.8 C24H38O4 –5
31.38 Bis[3,4-dichlorophenyl]sulfone 82.3 C12H6
Cl4
O2
S 3*
31.48 Bumetrizole 78.6 C17H18ClN3
O 57
31.51 Benz(a)anthracene-7,12-dione 76.1 C18H10O2 –48*
31.82 Bis(2-ethylhexyl) isophthalate 84.7 C24H38O4 –35
32.37 Decachlorobiphenyl 94.3 C12Cl10 –81
56
9
Figure 5. Compound misidentified by MassBank.us due to lack of the RI information. (A) MassBank.us hit. (B) NIST23 hit.
A
B
Table 4. Additional contaminants identified in drinking water using the Agilent GC/Q-TOF Pesticide PCDL.
RT Compound Name
Match
Factor Formula
6.17 2-Picoline 96.7 C6
H7
N
6.90 Methanesulfonate-methyl 79.6 C2
H6
O3
S
8.17 PPD/p-Phenylenediamine 80.0 C6
H8
N2
8.40 o-Toluidine 82.3 C7
H9
N
8.93 Thanite 83.9 C13H19NO2
S
9.17 Benzaldehyde 98.5 C7
H6
O
9.52 Phenol 89.6 C6
H6
O
11.46 Acetophenone 94.3 C8
H8
O
RT Compound Name
Match
Factor Formula
11.99 2,4,5-Trimethylaniline 82.7 C9
H13N
12.90 2-Nitrophenol 77.1 C6
H5
NO3
22.29 DPA/Diphenylamine (DFA) 84.7 C12H11N
22.44 Isoxadifen 93.3 C16H13NO3
24.64 Benzylbenzoate 83.0 C14H12O2
25.96 DIBP/Diisobutyl phthalate 86.1 C16H22O4
27.00 1-Methylphenanthrene 85.3 C15H12
57
10
Additionally, an interesting case was observed for a
compound with an RI of 1,858, whereby MassBank.us likely
provided correct ID (with the LMS of 85.8) while NIST23
did not (Figure 6), proving the value of including third-party
libraries in a compound identification workflow. The
compound’s most likely ID is thioxanthene, with a NIST23
experimental RI of 1,977, and an AI-predicted RI of 1,876.
The experimental RI for this compound, used for the NIST23
library search, provided a significant RI delta of 124 RI units.
However, the experimental RI for this compound was only
based on one data point, and thus may not be accurate. The
AI-predicted NIST23 RI generated a significantly smaller RI
delta (18 RI units). Due to the large RI difference between
the compound RI and the NIST23 experimental RI of
thioxanthene, another NIST23 hit, 1-methyldibenzothiophene
with the lower LMS of 81.1 was chosen (Figure 6B).
Among the contaminants with the highest response, which
were identified in drinking water extracts using all three
libraries, were mostly PAHs, DBPs, and phthalates (Figure 7).
Individual samples of drinking water from the same group
represented different households.
The contaminants in drinking water extracts were detected at
a wide range of concentrations, estimated to be from low- and
sub-ppb levels (for pesticides) to hundreds of ppb (in the case
of PAHs), suggesting that an extended dynamic range might
be desirable for this application.
Statistical analysis was performed in the MPP software,
where the differences between Weaverville and Irvine water
sources (n = 5 per group) were evaluated and displayed
on a volcano plot (Figure 8). The volcano plot displays fold
change versus statistical significance, and is used to quickly
detect differences between the two groups. Compounds
that were present in higher concentrations in Irvine water
compared to Weaverville are colored in red and shown in the
upper-right quadrant. Compounds that were found at higher
concentrations in Weaverville water extracts compared to
Irvine are colored in blue and displayed in the upper-left
quadrant. Most contaminants occurred at higher levels in
drinking water from Irvine (a more densely populated urban
area) compared to Weaverville.
Figure 6. Compound likely misidentified by NIST23 due experimental RI information available for only one data point. (A) MassBank.us hit. (B) NIST23 hit.
A
B
58
11
Figure 8. Comparison of water sourced in Irvine versus Weaverville on volcano plot, showing log2
of fold change (FC) versus
–log10 of p-value.
Figure 7. High-level contaminants identified in the drinking water of different households (n = 5 for each group) from Irvine (IR) and Weaverville (WV). Peak area
Chlorodibromomethane 9H-Fluoren-9-one Anthracene Dibutyl phthalate Fluoranthene Tributyl acetylcitrate
0
5
10
15
20
25
×105
IR
WV
59
www.agilent.com
DE50093873
This information is subject to change without notice.
© Agilent Technologies, Inc. 2023
Printed in the USA, December 5, 2023
5994-6966EN
Conclusion
Accurate mass libraries of environmental contaminants
(such as PFAS) broaden the scope of suspects screened
in environmental samples and increase confidence in the
identification of pollutants. The accurate mass library
described in this application note, containing over 150 PFAS
EI spectra, including several emerging volatile PFAS, enabled
identification of PFAS in drinking water samples using both
nontarget and target workflows.
Additional contaminants were identified in drinking water
from two different source categories, including disinfection
by-products, PAHs, pesticides, and other industrial
contaminants. Two drinking water sources were compared,
and a higher number of contaminants were identified in the
water extracts from Irvine compared to Weaverville.
Acknowledgement
The authors would like to thank the U.S. Environmental
Protection Agency (EPA) for preparing the PFAS standards
and providing them to Agilent Technologies through a
material transfer agreement.
References
1. Poly- and Perfluoroalkyl Substances (PFAS) Overview
and Current Activities. https://www.loudounwater.org/
residential-customers/facts-about-pfas
2. Hammer, J.; Endo, S. Volatility and Nonspecific van der
Waals Interaction Properties of Per- and Polyfluoroalkyl
Substances (PFAS): Evaluation Using Hexadecane/Air
Partition Coefficients. Environ. Sci. Technol. 2022 Nov 15,
56(22), 15737–15745. DOI: 10.1021/acs.est.2c05804
3. Liu, X. Understanding Semi-volatile Organic Compounds
(SVOCs) in Indoor Dust. Indoor Built Environ. 2022 Jan 10,
31(2), 291–298. DOI: 10.1177/1420326x211070859
4. Del Vento, S.; Halsall, C.; Gioia, R; Jones, K; Dachs, J.
Volatile Per- and Polyfluoroalkyl Compounds in the
Remote Atmosphere of the Western Antarctic Peninsula:
an Indirect Source of Perfluoroalkyl Acids to Antarctic
Waters? Atmos. Pollut. Res. 2012, 3(4), 450–455. DOI:
https://doi.org/10.5094/APR.2012.051
5. Wohlgemuth, G.; Mehta, S. S.; Mejia, R. F.; Neumann, S.;
Pedrosa, D.; Pluskal, T.; Schymanski, E. L.; Willighagen,
E. L.; Wilson, M.; Wishart, D. S.; et al. SPLASH, a Hashed
Identifier for Mass Spectra. Nat. Biotechnol. 2016, 34,
1099–1101. https://massbank.us/
6. Agilent ChemVista Library Manager. Agilent Technologies
technical overview, publication number 5994-5924EN,
2023.
7. Valdiviez, L.; Fiehn, O.; Nieto, S. Differences in Metabolic
Profiles of Individuals with Heart Failure Using
High-Resolution GC/Q-TOF. Agilent Technologies
application note, publication number 5994-6858EN, 2023.
8. Van Gansbeke, W.; Albertsdóttir, A. D.; Polet, M.;
Van Eenoo, P.; Nieto, S. Introducing Semi-Automated
GC/Q-TOF Screening with the AssayMAP Bravo Sample
Prep Platform for Antidoping Control. Agilent Technologies
application note, publication number 5994-6702EN, 2023.
9. Hanari, N.; Itoh, N.; Ishikawa, K.; Yarita, T.; Numata, M.
Variation in Concentration of Perfluorooctanoic
Acid in Methanol Solutions During Storage.
Chemosphere 2014 Jan, 94, 116–20. DOI: 10.1016/j.
chemosphere.2013.09.040
Application Note
Environmental
Author
Brooke C. Reaser
Agilent Technologies, Inc.
Abstract
Multiresidue pesticide analysis has become one of the most difficult but important
analytical challenges for those using gas chromatography and mass spectrometry.
The Agilent 8890 gas chromatograph (GC) coupled with the Agilent 7010 triple
quadrupole mass spectrometer (GC/TQ) with a high efficiency source 2.0 (HES 2.0)
upgrade is an analytically accurate, robust, and reproducible instrument for
multiresidue pesticide analysis of complex samples. The analysis of 190 pesticides
in a spinach extract was conducted using an Agilent QuEChERS extraction kit across
800 injections. Only GC inlet maintenance was required over the duration of the
injections. The instrument configuration that enabled robust performance included
a multimode inlet, a mid-column backflushing configuration, and the HES 2.0.
No degradation of the analytical method, sensitivity, or instrument performance
occurred, allowing for the high-throughput, accurate, robust, and sensitive detection
of pesticides in spinach.
Enhanced Longevity and
Revolutionized Robustness for the
Sensitive Detection of 190 Pesticides
over 800 Injections
Return to Table of Contents 61
2
Introduction
Multiresidue pesticide analysis remains
an important analytical challenge for
food safety.1-7 Pesticides used to improve
crop yield can end up in the final product,
raising concerns for consumer safety.
As a result, multiple governing bodies
worldwide have published requirements
for the maximum legal residue limit
(MRL) or tolerance for pesticides allowed
in a product. However, the number
of pesticides used in food products
continues to grow as novel chemicals
are introduced, which in turn increases
the complexity of the multiresidue
pesticide analysis.
For especially difficult matrices,
QuEChERS, which stands for quick,
easy, cheap, effective, rugged, and
safe, has become widely accepted as
a sample preparation technique for
multiresidue pesticide analysis.1
Agilent
QuEChERS extraction kits provide
prepackaged dispersive and extraction
products, extraction salts, and ceramic
homogenizers in easy-to-use kits. The
kits are ready-made for various methods,
including methods of the Association of
Official Agricultural Chemists (AOAC)2
and European Standard (EN).3
QuEChERS extracts of food commodities
can be analyzed by either GC or high
performance liquid chromatography
(HPLC) combined with a mass
spectrometer (MS) or tandem mass
spectrometers (MS/MS).4,5 Depending
on the extent of sample cleanup and
the food commodity being analyzed,
QuEChERS extracts can cause
contamination of the instrument,
resulting in poor data quality.6
This
contamination can exhibit as loss
of sensitivity, retention time shifting,
poor peak shape, and more. Regular
maintenance of the instrument, including
GC inlet maintenance, GC column
trimming, and ion source cleaning, is
required to ensure the robustness and
accuracy of the method results.
Backflushing is one of the key practices
in which GC/MS/MS analyses of
complex matrices can be improved.7
Backflushing refers to the reversing
of flows in the capillary column so
that unwanted matrix components
are flushed out of the GC split vent
instead of proceeding to the detector.
Backflushing can provide improved
method robustness and help minimize
the required maintenance of the
mass spectrometer.
Agilent has introduced the new HES 2.0
ion source as part of the 7010D triple
quadrupole mass spectrometer (TQ),
and it is also available as an upgrade
to 7010A/B/C GC/TQ instruments. The
HES 2.0 ion source provides improved
system robustness, allowing the analysis
of hundreds of injections of pesticides in
food matrices with only GC maintenance
and ion source cleaning necessary. The
HES 2.0 delivers the same unparalleled
analytical sensitivity for ultratrace-level
analysis as the original HES.
Multiresidue pesticide analysis in
spinach extract was carried out using
a 7010B GC/TQ upgraded with the
HES 2.0. Matrix-matched standards
were used to analyze and quantify over
400 injections of baby spinach extract
spiked with 50 ppb of multiresidue
standards, demonstrating both method
and instrument robustness. The
multimode inlet (MMI) and backflushing
between two 15 m columns allowed
for minimal downtime for GC inlet
maintenance. Sensitivity and quantitative
accuracy were maintained without
any maintenance performed on the
mass spectrometer.
Experimental
GC/TQ analysis
An 8890 GC with a 7010B TQ system
upgraded with the HES 2.0 was used for
analysis. The instrument and method
were configured as outlined in a previous
application note7
, as shown in Figure 1.
Agilent 7010
with HES 2.0
PSD
(helium)
Agilent 8890
GC
Liquid
Injector
Multimode
inlet
(helium)
HES 2.0
Agilent HP-5ms UI
15 m, 0.25 × 0.25
Figure 1. The Agilent 8890/7010B GC/TQ system upgraded with the HES 2.0 and system configuration.
62
3
The GC was equipped with an
Agilent 7650A automatic liquid
sampler (ALS) and 50-position tray.
The GC used an MMI to achieve a
temperature-programmed splitless
injection. Mid-column backflush was
carried out using an Agilent Purged
Ultimate Union (PUU) installed between
two identical 15 m columns; the 8890
GC pneumatic switching device (PSD)
module allowed for fewer occurrences
of regular maintenance. The method
parameters are listed in Table 1.
Table 1. Agilent 8890 GC and Agilent 7010B upgraded with the HES 2.0 ion source conditions for pesticide analysis.
GC
Instrument Agilent 8890 with Fast Oven, Auto Injector
and Tray
Inlet Multimode Inlet (MMI)
Mode Splitless
Purge Flow to Split Vent 15 mL/min at 0.75 min
Septum Purge Flow 3 mL/min
Septum Purge Flow Mode Switched
Injection Volume 1.0 µL
Injection Type Standard
L1 Air Gap 0.1 µL
Gas Saver Off
Inlet Temperature 60 °C for 0.1 min, then to 280 °C at 600 °C/min
Postrun Inlet Temperature 310 °C
Postrun Total Flow 25 mL/min
Carrier Gas Helium
Inlet Liner Agilent Ultra Inert 2 mm dimpled
liner (p/n 5190‑2297)
Oven
Initial Oven Temperature 60 °C
Initial Oven Hold 1 min
Ramp Rate 1 40 °C/min
Final Temperature 1 170 °C
Final Hold 1 0 min
Ramp Rate 2 10 °C/min
Final Temperature 2 310 °C
Final Hold 2 3 min
Total Run Time 20.75 min
Postrun Time (Backflushing) 1.5 min
Equilibration Time 3 min
Column 1
Type Agilent HP‑5ms UI (p/n 19091S‑431UI)
Length 15 m
Diameter 0.25 mm
Film Thickness 0.25 µm
Control Mode Constant flow
Flow 1.00 mL/min
Inlet Connection Multimode inlet (MMI)
Outlet Connection PSD (PUU)
PSD Purge Flow 5 mL/min
Postrun Flow (Backflushing) –7.873 mL/min
Column 2
Type Agilent HP‑5ms UI (p/n 19091S‑431UI)
Length 15 m
Diameter 0.25 mm
Film Thickness 0.25 µm
Control Mode Constant flow
Flow 1.200 mL/min
Inlet Connection PSD (PUU)
Outlet Connection MSD
Postrun Flow (Backflushing) 8.202 mL/min
MSD
Model Agilent 7010B
Source Agilent HES 2.0
Vacuum Pump Performance turbo
Tune File Atunes.eihs.tune.xml
Solvent Delay 3 min
Quad Temp (MS1 and MS2) 150 °C
Source Temperature 280 °C
Mode dMRM or Scan
He Quench Gas 4 mL/min
N2
Collision Gas 1.5 mL/min
MRM Statistics
Total MRMs (dMRM mode) 552
Minimum Dwell Time (ms) 2.63
Minimum Cycle Time (ms) 82.42
Maximum Concurrent MRMs 48
EM Voltage Gain Mode 10
63
4
The Agilent Pesticide and Environmental
Pollutant (P&EP) database (P&EP 4,
part number G9250AA) was used to
easily and rapidly create the dynamic
multiple reaction monitoring (dMRM)
method. This method, which enabled the
analysis of 190 pesticides with a total
of 552 MRMs, resulted in a maximum
of 48 concurrent MRMs, as shown in
Figure 2.
QuEChERS sample preparation
The sample preparation procedure is
summarized in Figure 3. A bag of frozen
organic baby spinach was homogenized
using a spice grinder. Then, eight
replicates were prepared. For each
replicate, 15 g of the homogenized
spinach were weighed into a 50 mL
test tube. Then, two of the replicates
were designated as samples, while the
remaining six were designated for pooled
matrix-matched standards. Fifteen
microliters of the internal standard
mixture (part number 5190-0502)
diluted to 50 ng/µL was added to the
two spinach samples. To all eight
replicates, 15 mL of 1% acetic acid in
acetonitrile was added and the mixture
was vortexed until well mixed. To each
Figure 2. Concurrent MRMs versus retention time.
Retention time (min)
Concurrent MRMs
0
4
8
12
16
20
24
28
32
36
40
44
48
52
4 5 6 7 8 9 10 11 12 13 14 15 16 17 18 19
Figure 3. Sample preparation workflow.
QuEChERS
extraction kit
Agilent
QuEChERS
dispersion kit
15 g of spinach
+ 15 mL of ACN with 1% AA,
vortex
Take 8 mL
supernatant
Shake and
centrifuge
Shake and
centrifuge
Dilute, spike, and
prepare in-vial
Sample analysis
on GC/TQ
64
5
50 mL tube, the salt packet and two
homogenizers from the QuEChERS kit
(part number 5982-5755) were added
for extraction. These were shaken for
1 minute, then centrifuged at 4,000 rpm
with a maximum radius of 17.4 cm
for 5 minutes. For the samples and
the pooled standards, 8 mL of the
supernatant was transferred to a
tube with salt for dispersion using the
QuEChERS kit (part number 5982-5058).
These were shaken for 30 seconds then
centrifuged as before for 5 minutes. The
supernatant for each of the samples
was removed and placed in an amber
glass vial. For the pooled standards,
the supernatant of all replicates was
removed and mixed in a large amber jar
for standard preparation.
Standard preparation
The multiresidue matrix-matched
standards were created from the
FDA analytical reference standards
kit (part number PSM-101). Mixes
A, B, C, D, E, L, M, N, O, and P from
the PSM-101 pesticides mix were
combined to create a 10 ppm stock
standard of 190 pesticide residues.
The stock solution was then diluted
in acetonitrile down to the following
nominal concentrations: 1,000, 100, 10,
and 1 ppb. In a GC vial, the standards
were combined with the spinach
extract. The internal standard mixture
of parathion-d10 and alpha-BHC-d6
, and
acetonitrile were combined until the
nominal concentration of the internal
standard was 50 ppb and the nominal
concentrations of the matrix-matched
standards in a total volume of 1,500 µL
were as follows: 0.1, 0.5, 1, 5, 10, 50,
100, 253.3, 500, and 1,000 ppb. Extra
vials of the 50-ppb matrix-matched
standard were made for quantification
to test robustness. The samples were
diluted by a factor of three in the vials
to match the matrix concentration in
the standards. This 3x dilution factor
was determined by analyzing the matrix
alone in full scan mode as described in a
previous application note7
to ensure that
the instrument was not overloaded or
saturated by the matrix. Vials with 250 µL
glass inserts were used with 100 µL
of each standard or sample in-vial for
GC analysis.
Sequence
Each sequence included 102 injections
of spinach extract, either as a sample
or matrix-matched standard. Additional
injections of blank acetonitrile were
used to evaluate system cleanliness
by ensuring no analyte carryover or
additional background contamination. A
representative MRM chromatogram of
the 50-ppb matrix-matched standards
in spinach extract is shown in Figure 4A
with a zoomed in portion shown in
Figure 4B. The sequence included:
– Matrix-matched calibration curve
(10 pts)
– Two spinach samples
– Matrix-matched calibration curve
(10 pts)
– Matrix-matched standards (50 ppb) ×
60 times
– Matrix-matched calibration curve
(10 pts) × 2 times, each standard in
duplicate
Figure 4. A representative chromatogram (A) and a zoomed in portion of the chromatogram (B).
×108
A
Acquisition time (min)
Counts
4 5 6 7 8 9 10 11 12 13 14 15 16 17 18 19
0
0.2
0.4
0.6
0.8
1.0
1.2
1.4
1.6
1.8
×107
B
Acquisition time (min)
Counts
5 6 7 8 9 10 11 12 13 14 15 16 17 18 19
0
0.1
0.2
0.3
0.4
0.5
0.6
0.7
0.8
0.9
1.0
65
6
To achieve more than 400 injections of
the 50 ppb matrix-matched standard,
seven sequences were run for a total
of 819 injections, of which 714 were
spinach matrix injections. After each
sequence, the GC inlet liner and septum
were changed, and the 5 µL syringe
was changed as necessary. Also, the
GC vials were refreshed with samples
and standards that had been stored in
the freezer. No additional instrument
maintenance was performed.
Results and discussion
Of the 190 pesticide residues analyzed
by GC/TQ, 114 were selected for further
study based on their analytical response
and performance. These 114 residues
were chosen because their calibration
curves were either linear or quadratic
throughout the seven sequences. These
curves did not require extensive analyst
intervention, such as manual integration,
and had calibration curves that
encompassed the 50 ppb point so that
the 50 ppb matrix-matched standards
for robustness could be calculated as
samples. Any points on the calibration
curve that had signal-to-noise (S/N) < 3,
were interfered with by contaminants,
or had accuracy greater than or equal to
± 25% were excluded (≥ ± 25%). A table
summarizing these residues can be
found at the end of the application note
in Table 2.
Many of the pesticide residues could
accurately be quantified down to 0.5
or 0.1 ppb while maintaining S/N > 10
and quantification accuracy of < 25%.
For example, Figures 5A, 5B, and 5C
show the 0.1 ppb peak, corresponding
qualifiers, and calibration curve of DCPA,
respectively. The data are defined well
by a quadratic calibration curve over
the calibration range 0.1 to 1,000 ppb
through four orders of magnitude.
DCPA has many MRLs as defined by the
US FDA down to 50 ppb in various fruits
and vegetables.
Figure 5. Integrated peak (A) and qualifiers (B) of the 0.1 ppb peak of DCPA as well as the full calibration
curve (C).
×103
×102
A
B
×10 C 1
Acquisition time (min)
Counts
+ MRM CID at 25.0 (298.9 & 221.0)
10.26 10.28 10.30 10.32 10.34 10.36 10.38 10.40 10.42 10.44
Acquisition time (min)
10.26 10.28 10.30 10.32 10.34 10.36 10.38 10.40 10.42 10.44
-0.2
0
0.2
0.4
0.6
0.8
1.0
1.2
1.4
1.6
1.8
2.0
10.325 min
-0.2
-0.1
0
0.1
0.2
0.3
0.4
0.5
0.6
0.7
0.8
0.9
1.0
1.1
298.9 & 221.0
300.9 & 223.0
331.8 & 300.9
Ratio = 89.2 (95.1%)
Ratio = 42.0 (97.0%)
DCPA
Relative concentration
0 1 2 3 4 5 6 7 8 9 10
-0.2
0
0.2
0.4
0.6
0.8
1.0
1.2
1.4
1.6
1.8
2.0
2.2
2.4
2.6
y = -0.013350x2
+ 2.835571x + 0.001611
R2
= 0.99899365
R = 0.99969055
Weight: 1/x
Relative abundance (%) Relative responses
66
7
Figures 6A and 6B show the chlorpyrifos
0.5 ppb peak and qualifiers (respectively),
while Figure 6C shows the corresponding
calibration curve. The curve is linear
through 3.5 orders of magnitude and
chlorpyrifos has MRLs in various food
commodities, the lowest of which is
0.01 ppm in food items such as egg, fig,
grape, and apple.
Figure 6. Integrated peak (A), qualifiers (B) of the 0.5 ppb peak of chlorpyrifos as well as the full calibration
curve (C).
×104
×102
A
B
×10 C 1
Acquisition time (min)
Counts
Relative concentration
Relative abundance (%) Relative responses -0.2
-0.1
0
0.1
0.2
0.3
0.4
0.5
0.6
0.7
0.8
0.9
1.0
1.1
196.9 & 169.0
198.9 & 171.0
313.8 & 257.8
Ratio = 103.0 (108.4%)
Ratio = 43.1 (98.7%)
+ MRM CID at 15.0 (196.9 & 169.0)
10.16 10.18 10.20 10.22 10.24 10.26 10.28 10.30 10.32
Acquisition time (min)
10.16 10.18 10.20 10.22 10.24 10.26 10.28 10.30 10.32
0.2
0.3
0.4
0.5
0.6
0.7
0.8
0.9
1.0
1.1
10.220 min Chlorpyrifos
0 1 2 3 4 5 6 7 8 9 10
-0.2
0
0.2
0.4
0.6
0.8
1.0
1.2
1.4
1.6
1.8
2.0
2.2
y = 2.182005x + 0.002352
R2
= 0.99501738
R = 0.99950640
Weight: 1/x2
67
8
In terms of spinach MRLs, Figures 7, 8,
and 9 provide three examples. Figure 7
shows the results for bifenthrin, which
is quadratic through 3.5 orders of
magnitude down to 0.5 ppb and has an
MRL of 0.2 ppm in spinach. Diazinon,
linear through 3.5 orders of magnitude
down to 0.5 ppb, is shown in Figure 8,
with an MRL in spinach of 0.7 ppm.
Figure 7. Integrated peak (A), qualifiers (B) of the 0.5 ppb peak of bifenthrin as well as the full calibration
curve (C).
×104
×102
A
B
×10 C 2
Acquisition time (min)
Counts
Relative concentration
Relative abundance (%) Relative responses
Bifenthrin
0 1 2 3 4 5 6 7 8 9 10
-0.2
0
0.2
0.4
0.6
0.8
1.0
1.2
1.4
1.6
1.8
2.0
2.2
2.4
y = 0.459107x2
+ 18.988674x + 0.012908
R2
= 0.99465789
R = 0.99640909
Weight: 1/x2
+ MRM CID at 25.0
(181.2 & 165.2)
14.14 14.16 14.18 14.20 14.22 14.24 14.26 14.28 14.30 14.32
Acquisition time (min)
14.14 14.16 14.18 14.20 14.22 14.24 14.26 14.28 14.30 14.32
2
3
4
5
6
7
8
14.202 min
-0.2
-0.1
0
0.1
0.2
0.3
0.4
0.5
0.6
0.7
0.8
0.9
1.0
1.1
181.2 & 165.2
181.2 & 166.2
166.2 & 165.2
Ratio = 87.4 (109.7%)
Ratio = 52.5 (107.2%)
68
9
Figure 8. Integrated peak (A), qualifiers (B) of the 0.5 ppb peak for diazinon as well as the full calibration
curve (C).
×103
×102
A
B
C
Acquisition time (min)
Counts
Relative concentration
Relative abundance (%) Relative responses
Diazinon
0 1 2 3 4 5 6 7 8 9 10
0
1
2
3
4
5
6
7
8 y = 0.638177x – 3.640200E-004
R2 = 0.98721937
R = 0.99294761
Weight: 1/x2
-0.1
0
0.1
0.2
0.3
0.4
0.5
0.6
0.7
0.8
0.9
1.0
1.1
137.1 & 84.0
199.1 & 93.0
Ratio = 64.7 (96.5%)
+ MRM CID at 10.0 (137.1 & 84.0)
8.44 8.46 8.48 8.50 8.52 8.54 8.56 8.58 8.60 8.62
Acquisition time (min)
8.44 8.46 8.48 8.50 8.52 8.54 8.56 8.58 8.60 8.62
0
0.5
1.0
1.5
2.0
2.5
3.0
3.5
4.0
4.5
8.523 min
69
10
Lastly, boscalid is shown in Figure 9
with an MRL of 1 ppb; it is easily defined
by a quadratic curve with four orders
of magnitude down to 0.1 ppb. In the
spinach sample, all three residues fell
below the limit of quantification (LOQ) of
the analytical method, and below their
respective spinach MRLs. In the spinach
samples, both boscalid and bifenthrin
fell below the limit of detection (LOD) as
no peak was detected for either residue.
However, a small amount of diazinon
was detected that was not present in
the solvent blank. However, the area of
the peak fell well below the LOQ and had
an S/N very close to 3, and therefore
approached or fell below the LOD.
×103
×102
×102
A
B
C
Acquisition time (min)
Counts
Relative concentration
Relative abundance (%) Relative responses
-0.1
0
0.1
0.2
0.3
0.4
0.5
0.6
0.7
0.8
0.9
1.0
1.1
140.0 & 112.0
140.0 & 76.0
111.9 & 76.0
Ratio = 87.0 (104.4%)
Ratio = 32.9 (99.3%)
+ MRM CID at 10.0 (140.0 & 112.0)
16.84 16.86 16.88 16.90 16.92 16.94 16.96 16.98 17.00 17.02
Acquisition time (min)
16.84 16.86 16.88 16.90 16.92 16.94 16.96 16.98 17.00 17.02
0
1
2
3
4
5
6
7
Boscalid 16.909 min
0 1 2 3 4 5 6 7 8 9 10
0
0.2
0.4
0.6
0.8
1.0
1.2
1.4
1.6
y = 0.635140x2
+ 8.900401x + 0.002796
R2
= 0.97434891
R = 0.99281390
Weight: 1/x2
Figure 9. Integrated peak (A), qualifiers(B) of 0.1 ppb peak of boscalid as well as the full calibration
curve (C).
70
11
The innovative HES 2.0 source enabled
enhanced response stability for the
analyzed pesticides over 400 replicate
injections of the matrix-matched
calibration standard at 50 ppb
analyzed within a sequence with over
800 total injections. Figure 10 shows
the 400 replicates of the 50 ppb
matrix-matched standard, calculated
as samples from the corresponding
calibration curves across six sequences.
The X-axis on the top corresponds to the
number of injections of just the 50 ppb
matrix-matched standard for robustness.
The lower X-axis corresponds to the total
injection number of spinach QuEChERS
matrix, and therefore excludes blank
injections. The concentration in ppb
versus injection number plot shows
how robust and accurate the analysis
was. The results showed all but one
point falling within ± 20% error, the
usual % error given by GC/TQ data, and
most points well within ± 10% of the
actual. The %RSD for 400 replicates
of all 114 residues are summarized
in Table 2, with nearly 80% of the 114
residues having %RSD < 10%, and only
five having %RSD above 20%. The
robustness of the analysis and the
instrument is clearly shown, with only
inlet maintenance required between
sequences and no further maintenance
of the instrument needed.
Figure 10. Calculated concentration of pesticides in 50 ppb matrix-matched standard over the course of
714 injections of spinach QuEChERS extract.
0 50 100 150 200 250 300 350 400
30
35
40
45
50
55
60
65
70
23 73 165 257 349 441 533 583 675
Adjusted 50 ppb spiked standard injection number
ppb
QuEChERS injection number
Parathion
BHC-alpha (benzene hexachloride)
Pentachlorobenzonitrile
Pentachlorothioanisole
Fenthion
71
12
Table 2. Summary of 114 residues, including calibration range, calibration curve fit, and %RSD over the 400 injections
of 50 ppb matrix-matched standard injections for robustness. Those results with %RSD > 20% are highlighted in red.
Name
Retention Time
(minutes)
Min Cal
(ppb)
Max Cal
(ppb) Curve Fit Curve Weight %RSD
Ethiolate 4.609 0.5 1,000 Linear 1/x2 7.6
Dichlorvos 4.826 0.5 1,000 Quadratic 1/x 10.4
Nicotine 5.424 0.5 1,000 Linear 1/x2 11.9
Biphenyl 5.615 1 1,000 Linear 1/x2 6.2
2‑Phenylphenol 6.457 5 1,000 Quadratic 1/x 7.5
Pentachlorobenzene 6.566 0.1 1,000 Linear 1/x2 4.8
Tecnazene 7.118 0.1 1,000 Linear 1/x2 3.9
Diphenylamine 7.186 0.1 1,000 Quadratic 1/x2 6.5
Ethoprophos 7.238 5 1,000 Quadratic 1/x 12.3
2,3,5,6‑Tetrachloroaniline 7.297 0.5 1,000 Linear 1/x2 4.9
Chlorpropham 7.325 0.5 1,000 Quadratic 1/x 7.5
Trifluralin 7.462 1 1,000 Quadratic 1/x2 6.6
Benfluralin 7.496 0.1 1,000 Quadratic 1/x2 7.2
BHC‑alpha (Benzene Hexachloride) 7.881 1 1,000 Linear 1/x2 1.0
2,6‑Diisopropylnaphthalene 8.02 5 1,000 Quadratic 1/x 5.5
Hexachlorobenzene 8.024 0.5 1,000 Quadratic 1/x 4.5
Ethoxyquin 8.034 0.1 1,000 Quadratic 1/x2 25.3
Dichloran 8.04 1 250 Linear 1/x2 7.7
Simazine 8.043 5 1,000 Linear 1/x2 7.0
Pentachloroanisole 8.073 0.1 1,000 Linear 1/x2 3.3
Atrazine 8.124 1 1,000 Linear 1/x2 5.5
Beta‑BHC 8.278 1 1,000 Linear 1/x2 4.9
Terbuthylazine 8.363 1 1,000 Linear 1/x2 14.6
BHC‑gamma (Lindane, Gamma HCH) 8.398 1 1,000 Linear 1/x2 4.5
Pentachloronitrobenzene 8.478 0.5 1,000 Quadratic 1/x2 33.7
Pentachlorobenzonitrile 8.515 0.1 1,000 Quadratic 1/x2 3.1
Diazinon 8.526 0.5 1,000 Linear 1/x2 5.8
Pyrimethanil 8.53 0.1 1,000 Linear 1/x2 5.0
BHC‑delta 8.763 1 1,000 Quadratic 1/x 11.4
Triallate 8.817 1 1,000 Quadratic 1/x2 4.4
Iprobenfos 8.942 0.5 1,000 Quadratic 1/x2 17.3
Pirimicarb 8.976 1 1,000 Linear 1/x2 8.9
Pentachloroaniline 9.178 0.1 1,000 Linear 1/x2 4.6
Propanil 9.193 0.5 1,000 Quadratic 1/x2 11.4
Metribuzin 9.256 0.5 1,000 Quadratic 1/x2 5.8
Dimethachlor 9.255 0.5 1,000 Linear 1/x2 6.0
Vinclozolin 9.372 0.1 1,000 Linear 1/x2 9.5
Chlorpyrifos‑methyl 9.404 0.1 1,000 Quadratic 1/x2 8.4
Parathion‑methyl 9.403 5 1,000 Quadratic 1/x 9.3
Ametryn 9.495 0.5 1,000 Quadratic 1/x2 6.5
Tolclofos‑methyl 9.496 5 1,000 Linear 1/x2 5.8
Prometryn 9.541 0.1 1,000 Quadratic 1/x2 6.9
Pirimiphos‑methyl 9.85 0.5 1,000 Quadratic 1/x2 8.9
Fenitrothion 9.855 0.1 1,000 Quadratic 1/x2 10.2
72
13
Name
Retention Time
(minutes)
Min Cal
(ppb)
Max Cal
(ppb) Curve Fit Curve Weight %RSD
Ethofumesate 9.877 0.5 1,000 Quadratic 1/x2 6.5
Malathion 9.995 10 1,000 Quadratic 1/x 20.0
Pentachlorothioanisole 10.032 0.1 1,000 Linear 1/x2 3.5
Metolachlor 10.166 5 1,000 Linear 1/x2 6.2
Fenthion 10.187 0.5 1,000 Linear 1/x2 2.4
Chlorpyrifos 10.224 0.5 1,000 Linear 1/x2 4.5
Parathion 10.242 1 1,000 Linear 1/x2 2.7
Triadimefon 10.275 0.5 1,000 Quadratic 1/x 11.6
Tetraconazole 10.319 0.5 1,000 Quadratic 1/x2 7.5
DCPA (Dacthal, Chlorthal‑dimethyl) 10.328 0.1 1,000 Quadratic 1/x 7.3
Isocarbophos 10.346 0.1 1,000 Linear 1/x2 4.7
Butralin 10.496 0.5 1,000 Linear 1/x2 5.7
Cyprodinil 10.672 0.5 1,000 Linear 1/x2 7.8
MGK‑264 10.709 0.1 1,000 Linear 1/x2 7.3
Pendimethalin 10.797 0.5 1,000 Linear 1/x2 4.6
Penconazole 10.826 0.5 1,000 Quadratic 1/x2 9.8
Heptachlor Exo‑epoxide 10.904 10 1,000 Quadratic 1/x 14.1
Fipronil 10.915 0.5 1,000 Quadratic 1/x 10.7
Triadimenol 11.008 0.5 1,000 Quadratic 1/x2 7.1
Quinalphos 11.01 1 1,000 Linear 1/x2 3.3
Chlordane‑trans 11.326 100 1,000 Quadratic 1/x 13.6
DDE‑o,p' 11.363 0.1 500 Quadratic 1/x2 11.8
Mepanipyrim 11.458 0.5 1,000 Linear 1/x2 7.1
Flutriafol 11.596 0.5 1,000 Quadratic 1/x 6.6
Flutolanil 11.664 0.5 1,000 Quadratic 1/x2 4.6
Napropamide 11.697 0.5 1,000 Linear 1/x2 8.5
Hexaconazole 11.73 0.5 1,000 Linear 1/x2 8.6
Isoprothiolane 11.776 0.1 1,000 Linear 1/x2 4.8
Prothiofos 11.782 0.5 1,000 Linear 1/x2 5.2
Fludioxonil 11.819 0.5 1,000 Quadratic 1/x2 6.9
DEF 11.871 0.5 1,000 Linear 1/x2 7.4
DDE‑p,p' 11.91 0.1 500 Quadratic 1/x2 9.8
Oxyfluorfen 11.988 0.5 1,000 Linear 1/x2 5.2
Myclobutanil 12.013 0.5 1,000 Quadratic 1/x2 8.2
Buprofezin 12.066 1 1,000 Quadratic 1/x2 6.2
Bupirimate 12.089 0.5 1,000 Linear 1/x2 6.7
Kresoxim‑methyl 12.093 0.5 1,000 Quadratic 1/x 5.9
Chlorfenapyr 12.326 0.5 1,000 Quadratic 1/x2 4.8
Endrin 12.425 5 1,000 Quadratic 1/x 10.3
Ethion 12.718 5 1,000 Linear 1/x2 7.5
Benalaxyl 13.167 0.5 1,000 Quadratic 1/x2 7.7
Trifloxystrobin 13.223 0.5 1,000 Linear 1/x2 5.3
Quinoxyfen 13.222 0.1 1,000 Linear 1/x2 7.7
Endosulfan Sulfate 13.328 1 1,000 Quadratic 1/x2 13.2
Tebuconazole 13.565 0.5 1,000 Quadratic 1/x2 6.6
Nuarimol 13.595 0.5 1,000 Quadratic 1/x2 6.4
Triphenyl Phosphate 13.659 0.5 1,000 Quadratic 1/x2 6.1
73
14
Name
Retention Time
(minutes)
Min Cal
(ppb)
Max Cal
(ppb) Curve Fit Curve Weight %RSD
Piperonyl butoxide 13.662 0.5 1,000 Quadratic 1/x2 5.9
Epoxiconazole 13.876 0.5 1,000 Quadratic 1/x2 8.5
Spiromesifen 14.014 1 1,000 Quadratic 1/x 15.5
Tetramethrin I 14.207 0.5 1,000 Quadratic 1/x 7.4
Bifenthrin 14.179 0.5 1,000 Quadratic 1/x2 4.4
EPN 14.226 10 1,000 Quadratic 1/x2 5.8
Bromopropylate 14.221 0.5 1,000 Quadratic 1/x2 5.2
Etoxazole 14.375 0.5 1,000 Quadratic 1/x2 6.3
Tebufenpyrad 14.398 0.5 1,000 Quadratic 1/x2 6.9
Fenamidone 14.449 0.5 1,000 Quadratic 1/x2 7.4
Tetradifon 14.72 5 1,000 Quadratic 1/x2 9.2
Metrafenone 15.648 0.5 1,000 Quadratic 1/x2 6.4
Bitertanol I 15.857 0.5 1,000 Quadratic 1/x2 9.7
Spirodiclofen 15.976 5 1,000 Quadratic 1/x2 49.3
Pyridaben 16.081 0.5 1,000 Quadratic 1/x2 5.8
Cyfluthrin I 16.484 5 1,000 Quadratic 1/x2 22.2
Fenbuconazole 16.535 5 1,000 Quadratic 1/x2 9.5
Cypermethrin I 16.8 5 1,000 Quadratic 1/x 23.8
Boscalid 16.909 0.1 1,000 Quadratic 1/x2 8.4
Ethofenprox 17.096 0.5 1,000 Quadratic 1/x2 5.9
Difenoconazole I 18.148 1 1,000 Quadratic 1/x 12.4
Azoxystrobin 18.787 10 1,000 Quadratic 1/x2 11.2
Dimethomorph I 19.175 5 1,000 Quadratic 1/x 15.3
74
www.agilent.com
DE34182072
This information is subject to change without notice.
© Agilent Technologies, Inc. 2024
Printed in the USA, May 3, 2024
5994‑7385EN
Conclusion
The analytical performance of the
Agilent 7010 Series triple quadrupole
mass spectrometer (GC/TQ) upgraded
with the HES 2.0 electron ionization
(EI) source was demonstrated for
multiresidue pesticide analysis. The
system demonstrates analytical
sensitivity as the same or better
than the original HES as well as
excellent accuracy and robustness.
The 7010 GC/TQ with HES 2.0,
coupled with an Agilent 8890 GC
with an MMI inlet and 15 m × 15 m
mid-column backflush configuration
minimizes instrument downtime
by allowing for inlet maintenance
without requiring the cooling of the
heated zones. With no impact to the
analytical method or degradation
of the instrument performance, this
application demonstrates the ability of
the instrument to provide robust and
reliable analytical results, including for
challenging matrices.
References
1. Anastassiades, M.; Lehotay, S. J.;
Stajnbaher, D.; Schenck, F. J. Fast and
Easy Multiresidue Method Employing
MeCN Extraction/Partitioning and
“Dispersive Solid-Phase Extraction”
for the Determination of Pesticide
Residues in Produce. J. AOAC Int.
2003, 86, 412− 431.
2. Pesticide Residues in Foods by
MeCN Extraction and Partitioning
with Magnesium Sulfate. Official
Methods of Analysis of AOAC
International; AOAC International:
Gaithersburg, MD, 2007; Method
2007.1.
3. Lehotay, S. J. QuEChERS Sample
Preparation Approach for Mass
Spectrometric Analysis of Pesticide
Residues in Foods. Methods
Mol. Biol. 2011, 747, 65–91. doi:
10.1007/978-1-61779-136-9_4.
PMID: 21643905.
4. Alder, L.; Greulich, K.; Kempe, G.;
Vieth, B. Residue Analysis of 500
High Priority Pesticides: Better
by GC-MS or LC–MS/ MS? Mass
Spectrom. Rev. 2006, 25, 838–865.
5. Chamkasem, N.; Ollis, L. W.;
Harmon, T.; Lee, S.; Mercer, G.
Analysis of 136 Pesticides
in Avocado Using a Modified
QuEChERS Method with LC-MS/MS
and GC-MS/MS. J. Agric. Food Chem.
2013, 61(10), 2315–2329. DOI:
10.1021/jf304191c
6. Lehotay, S. J.; Han, L.;
Sapozhnikova, Y. Automated
Mini-Column Solid-Phase Extraction
Cleanup for High-Throughput
Analysis of Chemical Contaminants
in Foods by Low-Pressure Gas
Chromatography-Tandem Mass
Spectrometry. Chromatographia
2016, 79(17), 1113–1130. DOI:
10.1007/s10337-016-3116-y.
7. Andrianova, A.; Zhao, L. Five Keys to
Unlock Maximum Performance in
the Analysis of Over 200 Pesticides
in Challenging Food Matrices by
GC/MS/MS. Agilent Technologies
application note, publication number
5994-4965EN, 2022.
Application Note
Food
Authors
Anastasia A. Andrianova and
Limian Zhao
Agilent Technologies, Inc.
Abstract
This application note presents results for the sensitive and robust quantitation of
246 pesticides in black tea extract with the Agilent 7010D Triple Quadrupole Mass
Spectrometer (GC/TQ) featuring a second-generation High Efficiency Source 2.0
(HES 2.0), that addresses the challenges posed by residual pesticide analysis in
complex matrices. By optimizing sample preparation and using state-of-the-art
GC/MS hardware, including ion source technology and midcolumn backflushing,
excellent calibration performance and sensitivity at low-ppb levels were achieved.
The method demonstrated exceptional ruggedness and robustness over
800 consecutive injections of a black tea extract spiked with pesticides at 2 ppb, with
high precision and low RSDs, ensuring prolonged instrument uptime and maximum
throughput. The demonstrated limits of quantitation (LOQs) were as low as 0.01 ppb
for over a third of evaluated compounds, and the calibration range spanned up to
five orders of magnitude while meeting SANTE 11312/2021 guidelines.
This application note highlights intelligent GC/TQ features, such as early
maintenance feedback and an instrument health status dashboard, maintaining
confidence in the results for a high-throughput analysis. The updated data
acquisition platform provides enhanced user experience, including a new
implementation of the retention time locking functionality.
Brewing Excellence: Quantitating
Over 200 Pesticides in Black Tea with
Steady Performance and Maximized
Uptime by GC/MS/MS
76 Return to Table of Contents
2
Introduction
Tea is among the most common nonalcoholic beverages
consumed worldwide. Like many foods, tea cultivation relies
heavily on pesticide application to combat pests, leading to
concerns over pesticide residues intensifying.1
Assessing pesticide levels in tea is essential for evaluating
safety, and is required by many regulatory bodies, including
the European Commission and the US Environmental
Protection Agency.2,3 A complete workflow for pesticide
testing in tea includes sample extraction via QuEChERS,
followed by extract cleanup, and subsequent testing with
liquid and gas chromatography coupled with triple quadrupole
mass spectrometry (LC/TQ and GC/TQ).4
Workflow
performance should enable sufficient method sensitivity,
calibration range, pesticide recovery from the extraction,
and precision. Sensitivity requirements are set based on
the maximum residue levels (MRLs), which are the highest
levels of pesticide residue that are legally allowed in or on
food or feed when pesticides are applied correctly. The ability
to calibrate over a wide dynamic range allows the varying
MRLs for individual compounds monitored in the commodity,
which can vary from 10 ppb to 100 ppm. When a specific
pesticide lacks an established MRL, a default limit of 10 ppb
is commonly applied. Efficiency of extraction and cleanup
are characterized in terms of recovery of matrix spikes, and
precision is expressed in terms of relative standard deviation
(RSD) of repeat analyses.
This application note presents a complete GC/TQ workflow
solution for the accurate and reliable analysis of 246 volatile
and semivolatile pesticides in black tea. Excellent analytical
performance of the workflow was achieved through a
combination of cutting-edge technology and optimized
methodology that included:
– Sample preparation using QuEChERS extraction, followed
by EMR mixed-mode pass-through cleanup using
Agilent Captiva EMR–GPD cartridges
– Agilent 8890 GC hardware and GC supplies
– Novel electron ionization (EI) source technology with
HES 2.0
– Built-in GC/TQ MS intelligence and new software
functionality for method setup, maintenance, and system
health evaluation
The presented workflow allowed for quantitating
246 pesticide residues in black tea with LOQs as low as
0.01 ppb for 34% of the targets, at or below 0.1 ppb for 74%
of compounds, and below 2 ppb for 96%. Matrix-matched
calibrations demonstrated excellent accuracy over a wide
dynamic range, spanning up to five orders of magnitude
over 0.01 to 1,000 ppb in the complex black tea extract.
Method ruggedness was demonstrated through maintaining
measurement accuracy with good precision (RSDs < 20%
for 176 compounds) for black tea extract spiked at 2 ppb
sequentially analyzed over 800 runs spanning 17 days of
continuous analysis. The new HES 2.0 ion source is equipped
with a novel dipolar radiofrequency (RF) lens that redirects
the carrier gas ions and, as a result, enables improved
system robustness and maximizes uptime while maintaining
unparalleled analytical sensitivity.
Experimental
GC/TQ analysis
The 8890 GC and 7010D GC/TQ systems (Figure 1A) were
used and configured to achieve the best sensitivity, maintain
a wide calibration range, and provide the most rugged
method performance. The GC was configured with the Agilent
7693A automatic liquid sampler (ALS) and 150-position
tray. The system used a multimode inlet (MMI) operated
in temperature-programmed splitless injection mode (also
known as cold splitless). The injection parameters were
optimized for maximizing sensitivity while limiting carryover.
Midcolumn backflush capability was provided by the Agilent
Purged Ultimate Union (PUU) installed between two identical
15 m columns, and the Agilent 8890 pneumatic switching
device (PSD) module (Figure 1B). The instrument operating
parameters are listed in Table 1.
Data were acquired in dynamic MRM (dMRM) mode, which
provides the capability for large multi-analyte assays and the
accurate quantitation of narrow peaks by an automatically
determined most-efficient dwell time distribution. The dMRM
capability enabled successful analysis for a large panel
of 246 pesticides, with 749 total MRM transitions with up
to 64 concurrent MRMs. Furthermore, dMRM allows the
analyst to add and remove additional analytes with ease. The
acquisition method was retention time locked to match the
retention times in the Agilent MassHunter Pesticides and
Environmental Pollutants MRM Database 4.0 (P&EP 4.0)5
,
77
3
Figure 1. The Agilent 8890 GC system with the Agilent 7010D GC/TQ system (A) and system configuration (B).
PSD
(helium)
Agilent 8890 GC
Liquid
Injector
Multimode
inlet (helium)
Agilent 7010D TQ MS
HES 2.0
Agilent HP-5ms UI,
15 m × 0.25 mm,
0.25 µm
Agilent HP-5ms UI,
15 m × 0.25 mm,
0.25 µm
A B
which was used to seamlessly create the MS method. The
use of P&EP 4.0 increased the ease and speed of setting up
a targeted dMRM method. High method selectivity in the
presence of coeluting matrix components was achieved by
selecting the best MRM transitions from up to nine transitions
available for each compound in the P&EP 4.0 database.
Three targets were not available in the P&EP 4.0 database
(flonicamid, bioallethrin, and cycloxydim). For these
compounds, MRM transitions were developed using
Agilent MassHunter Optimizer for GC/TQ, operating in Start
from Full Scan mode. The Optimizer is fully integrated into
MassHunter Acquisition 13.0 for GC/MS (Figure 2).
The acquisition method was retention time locked to
the P&EP database with chlorpyrifos-methyl eluting at
9.143 minutes. The retention time locking functionality,
integrated in MassHunter Acquisition 13.0 for GC/MS, has
an updated user-friendly and intuitive interface (Figure 3). It
allows for semi-automated or manual compound selection,
provides a choice to use three or five points for retention time
locking calibration, and features both a visual and quantitative
assessment of the calibration curve fit, while providing the
tools to maintain excellent precision of retention times, even
after column trimming.
Full scan data acquisition mode was used for the initial
screening of the matrix extract. This screening was used
to evaluate in-source loading, and for monitoring the
efficiency of the sample cleanup procedure that followed
QuEChERS extraction.
Agilent MassHunter Workstation software, including Agilent
MassHunter Acquisition 13.0 for GC/MS, MassHunter
Quantitative Analysis 12.1, and MassHunter Qualitative
Analysis 12.0 packages were used in this work.
78
4
Table 1. Agilent 8890 GC system with Agilent 7010D gas chromatograph and mass spectrometer conditions for pesticide analysis.
Parameter Value
GC Agilent 8890 with fast oven, auto injector and tray
Inlet MMI
Mode Cold splitless
Purge Flow to Split Vent 60 mL/min at 3 min
Septum Purge Flow 3 mL/min
Septum Purge Flow Mode Switched
Injection Volume 1.0 µL
Injection Type Reversed 2-layer (L2, L1)
L1 Airgap 0.2 µL
L2 Volume (ISTD) 0.2 μL
L2 Airgap 0.2 μL
Gas Saver On at 30 mL/min after 5 min
Inlet Temperature 60 °C for 0.1 min, then to 280 °C at 600 °C/min,
hold for 5 min, then to 325 °C at 600 °C/min
Postrun Inlet Temperature 310 °C
Postrun Total Flow 25 mL/min
Carrier Gas Helium
Inlet Liner Agilent Ultra Inert 2 mm dimpled liner
(p/n 5190-2297)
Oven
Oven Program
60 °C for 1 min;
40 °C/min to 170 °C;
Hold 0 min;
10 °C /min to 310 °C;
Hold 2.25 min
Total Run Time 20 min
Postrun Time 1.5 min
Equilibration time 0.5 min
Column 1
Type Agilent HP-5ms UI, 15 m × 0.25 mm, 0.25 µm
(p/n 19091S-431UI-KEY)
Control Mode Constant flow
Flow 1.0 mL/min (then retention time locked)
Inlet Connection MMI
Outlet Connection PSD (PUU)
PSD Purge Flow 5 mL/min
Postrun Flow (Backflushing) –7.873
Parameter Value
Column 2
Type Agilent HP-5ms UI, 15 m × 0.25 mm, 0.25 µm
(p/n 19091S-431UI-KEY)
Control Mode Constant flow
Flow 1.2 mL/min (then retention time locked)
Inlet Connection PSD (PUU)
Outlet Connection MSD
Postrun Flow (Backflushing) 8.202
MSD
Model Agilent 7010D
Source HES 2.0
Vacuum Pump Performance turbo
Tune File atunes.eihs2.jtune.xml
Solvent Delay 3.75 min
Quad Temperature
(MS1 and MS2) 150 °C
Source Temperature 280 °C
Mode dMRM or Scan
He Quench Gas 2.25 mL/min
N2
Collision Gas 1.5 mL/min
MRM Statistics
Total MRMs (dMRM mode) 749
Minimum Dwell Time 5.42 ms
Minimum Cycle Time 85.01 ms
Maximum Concurrent MRMs 64
EM Voltage Gain Mode 10
Scan Parameters
Scan Type MS1 Scan
Scan Range 45 to 450 m/z
Scan Time (ms) 220
Step Size 0.1 amu
Threshold 0
EM Voltage Gain Mode 1
79
5
Figure 2. Agilent MassHunter Optimizer software for GC/TQ, used for the automated development of MRM transitions.
80
6
Figure 3. New Agilent retention time locking software in Agilent MassHunter Acquisition 13.0 for GC/MS.
81
7
Sample preparation
Black tea powder was obtained from a local grocery store.
Black tea powder (2 g) was extracted with a modified
QuEChERS extraction using acetonitrile (ACN) with 2% formic
acid and EN extraction salt. The crude tea extract then was
mixed with 2% of acidic buffer. The sample mixture was
cleaned by EMR mixed-mode pass-through cleanup using
Agilent Captiva EMR–GPD 6 mL. The sample eluent was dried
with anhydrous MgSO4
to remove water residue completely
before GC/MS/MS analysis. The sample preparation
procedure flowchart is shown in Figure 4, and details will be
discussed in a separate application note. The entire sample
preparation procedure resulted in a 5x dilution factor.
Pesticide standards
Agilent GC pesticide standards 1 through 12
(part numbers PSM-100-A through -L) and Agilent GC/LC
pesticide standards 1, 2, and 3 (part numbers PSM-100-AA,
PSM-100-AB, PSM-100-AC) were used for preparing
matrix matched calibration standards. A combination of
the 15 used standards yielded a mix of 246 pesticides
commonly regulated by the FDA, USDA, and other global
governmental agencies.
Figure 4. QuEChERS sample preparation and cleanup method for black tea.
2 g of
black tea Agilent
QuEChERs EN
extraction kit
Mechanical
shaker
Centrifuge
– Dry sample hydration
and equilibration
– Addition of extraction
solvent
Sample cleanup
Sample extraction
Black tea extract on
Agilent Captiva
EMR–GPD
Sample eluent
drying with MgSO4
Sample analysis
on the Agilent
7010D GC/TQ
Crude extract
2 g of
– Premixing with
2% acidic buffer
– Loading 2.5 to
3 mL on cartridge
82
8
Matrix-matched calibration
Calibration performance was evaluated using a series of
matrix matched calibration standards, ranging from 0.01 to
1,000 ppb, including 0.01, 0.05, 0.1, 0.5, 1, 2, 5, 10, 50, 100,
200, 500, and 1,000 ppb. The standard parathion-d10 (Agilent
QuEChERS IS standard number 6, part number PPS-610-1)
was used as the internal standard for quantitation of the
target pesticides. It was added at 0.2 μL through reversed
sandwich injection with the ALS to a final concentration of
10 ppb in the injected sample.
An appropriate calibration function, either linear or quadratic,
guided by the lower value of the relative standard error (RSE)
was used. A weighting factor of 1/x allowed for maintaining
accuracy across the entire calibration range. The deviation
of the back-calculated concentrations of the calibration
standards from the true concentrations, using the calibration
curve in the relevant region, did not exceed ± 20%.
The concentrations expressed in ppb (w:v) correspond to
the pesticide concentration in the injected sample. The
QuEChERS sample preparation procedure described in the
Sample preparation section resulted in a dilution factor of 5.
Hence, the concentrations measured in the injected samples
were five times lower than the corresponding concentration in
the black tea sample, expressed in µg/kg.
Analyte protectants were not used in this work. The
preliminary investigation showed that analyte protectants
did not have a response enhancement effect on most of the
compounds when analyzed in the rich and complex black tea
matrix. It is of note that analyte protectants often significantly
enhance target analyte response and stability as is described
in-depth in the peer-reviewed literature.6
Recovery evaluation
Sample preparation efficiency was evaluated by performing
recovery studies. The surrogate black tea matrix was spiked
at two different levels, 10 and 50 ppb, with six replicates
at each level. The samples were extracted and cleaned up.
Assuming 100% recovery, pesticide concentrations in the
prespiked samples were expected to be 2 and 10 ppb due to
a 5x dilution rate. A blank black tea extract was postspiked
with the pesticide standard to achieve final concentrations
of 2 and 10 ppb. The prespiked and postspiked samples
were analyzed, and the response areas were compared.
The recovery was measured as the ratio of the pesticide
peak area in the prespiked sample to the area in the
postspiked samples.
Results and discussion
As contemporary GC/MS technology continues to advance,
so do the expectations for high sample throughput, intuitive
user-friendly system setup and configuration, and streamlined
maintenance. The demands for enhanced analytical
performance are driven by the evolving regulations in
pesticide residue analysis and food safety.
Several best practices to achieve the best GC/TQ
performance in pesticide residue analyses were described in
Agilent application note 5994-4965EN.7
This work presents a
complete workflow for analyzing 246 pesticides in black tea,
while implementing the previously described best practices
and offering further method and technology enhancements.
The innovative HES 2.0 yielded enhanced GC/TQ performance
stability, as evidenced by precise results at the low
concentration of 2 ppb over 800 consecutive injections of
complex black tea extract.
The technology and method enhancements that enable
unparalleled GC/TQ performance while ensuring stable and
reliable results in a high-throughput setting are outlined
in this application note and grouped into four categories:
sample preparation, GC instrumentation and supplies, MS
electron ionization technology advancements, and instrument
intelligence and software functionality.
Effective matrix cleanup
Sample preparation is a key component of performing
successful pesticide analysis. Performing analysis of
samples prepared by QuEChERS extraction, particularly
when analyzing complex pigmented samples such as black
tea without adequate cleanup, can lead to increased system
maintenance. The parts of the system that are affected
without adequate sample cleanup include liner replacement,
GC column trimming, and inlet and MS source cleaning. As
a result, throughput is decreased. Further, the presence of
large amounts of matrix can affect the accuracy of results,
often most pronounced with difficult to analyze pesticides.
The EMR mixed-mode pass-through cleanup using Captiva
EMR with Carbon S cartridges is a simplified procedure
that demonstrates an improvement on both sample matrix
removal, and overall recovery and reproducibility of targets.
As shown in Figure 5, the abundance of the TIC signal in full
scan data acquisition mode was noticeably reduced for black
tea extract after cleanup when comparing the crude extracts
before cleanup.
83
9
Performing matrix screening in full scan data acquisition
mode, as shown in Figure 5, facilitates the evaluation of
in-source matrix loading, as discussed in 5994-4965EN.7
Every MS source has a limitation on the amount of material
present in the source, at any point in time, to maintain optimal
performance. Quantitation accuracy of the analysis can
be significantly compromised if the source is overloaded
with matrix.
Hence, it is essential to analyze the matrix in full scan
mode to evaluate the TIC and maintain optimal GC/TQ
performance. For best performance with the HES 2.0 source,
it is recommended to have a TIC full scan abundance below
7 × 107
counts when analyzing with an EM gain set to 1. As
shown in Figure 5, black tea extract is complex, featuring
abundant matrix components. Cleaning up the extract is key
to lowering the matrix background that leads to adequate
in-source loading, enhancing selectivity and sensitivity,
widening the dynamic range, and allowing for less frequent
system maintenance, increasing productive uptime.
GC instrumentation and supplies
Midcolumn backflushing: The Agilent 8890 GC provides an
easy-to-use midcolumn backflushing functionality that results
in increased sample throughput through shorter analysis
times and less frequent column maintenance.
Midcolumn backflush allows for the elution of the high
boiling point matrix components from the column in a
shorter time and without eluting high-boiling matrix into
the MS. Midcolumn backflushing is a technique in which
the carrier gas flow is reversed after the last analyte has
exited the column and all MS data are collected. The oven is
then held at the final temperature in postrun mode, with the
reversed carrier gas flow through the first column. The high
boilers are eluted back out the head of the column and into
the split vent trap. The ability to reverse the flow is provided
by the PUU. The PUU is a tee that is inserted, in this case,
between two identical 15 m columns. During the analysis,
a small makeup flow of carrier gas from the 8890 PSD is
used to sweep the connection. During backflushing, the
Figure 5. Scan TIC of black tea extract. The green trace corresponds to matrix sample with Agilent Captiva-EMR cleanup, and the red trace corresponds to matrix
sample without cleanup.
0
0.1
0.2
0.3
0.4
0.5
0.6
0.7
0.8
0.9
1.0
1.1
1.2
1.3
1.4
1.5
1.6
1.7
1.8
1.9
2.0
2.1
2.2
2.3
2.4
2.5
2.6
2.7
2.8
2.9
3.0
3.1
3.2
3.3
3.4
3.5
4 5 6 7 8 9 10 11 12 13 14 15 16 17 18 19
15 min ×108
Acquisition time (min)
Counts
Extract before cleanup
After cleanup
84
10
makeup flow from the PSD is raised to a much higher value,
sweeping high boilers backward out of the first column while
simultaneously providing forward flow in the second column.
For the configuration in this application, the backflushing
time was 1.5 minutes. More details about using PSD for
backflushing in the Agilent 8890 GC system can be found in
Agilent application note 5994-0550EN.8
Figure 6 illustrates the effectiveness of the backflush
technique in reducing cycle time without carryover of the
black tea matrix. The cycle time was reduced by 50% and the
columns did not have to be exposed to the higher bake-out
temperatures for an extended time. Using backflushing,
excess column bleed and heavy residues are not introduced
into the MSD, thereby reducing ion source contamination.
1
1
5 6 7 8 9 10 11 12 13 14 15 16 17 18 19 20 21 22 23 24 25 26 27 28 29 30 31 32 33 34 35 36 37 38 39
It took an additional 20 minutes with the columns
heated to 310 °C to remove these high boilers
Run stopped at 20 minutes
and backflushed for 1.5 minutes
The following blank has no carryover peaks
Run stopped at 20 minutes,
no backflushing, no bakeout
The following blank has carryover peaks
Siloxanes from the septum
Siloxanes from the septum
Acquisition time (min)
Figure 6. TIC Scan chromatograms of black tea extract, followed by analysis of an instrument blank with: column bake-out, with backflush, and without backflush
or bake-out.
85
11
The backflush setup process has been simplified with the
introduction of new tools that allow for making a capillary
flow technology (CFT) connection with ease. These tools
include the gold-plated flexible metal ferrules (part number
G2855-28501) and the GC column installation preswaging
tool for Flexible Metal ferrules into Capillary Flow Technology
devices (part number G3440-80227)—shown in Figure 7.
Figure 7. Flexible metal ferrules (part number G2855-28501) (A) and the GC
column installation preswaging tool for Flexible Metal ferrules into Capillary
Flow Technology devices (part number G3440-80227) (B).
A B
Additionally, MassHunter Acquisition 13.0 for GC/MS provides
intuitive guides for backflushing setup and review. Figure 8
shows the backflush overview tab in the GC Method Editor in
MassHunter Acquisition 13.0 for GC/MS.
Figure 8. Backflush summary in Agilent MassHunter Acquisition 13.0 for GC/MS.
86
12
GC injection optimization: Efficiently volatilizing the sample
in the GC inlet is an essential component of successful
GC/MS analysis. Various sample introduction techniques are
aimed at preserving thermally labile and active compounds.
In this work, cold splitless and solvent vent injection modes
were evaluated.
As shown on the left in Figure 9, the use of solvent vent mode
for analyzing black tea extract resulted in very large caffeine
carryover into the subsequent analyses. To reduce caffeine
carryover, cold splitless injection mode was used (Figure 9,
at right). Increase of the splitless purge time to 3 minutes
resulted in enhanced method sensitivity without deteriorating
the chromatographic peak shape for the targets.
0
1
2
3
4
5
0
1
2
3
4
5
4 5 6 7 8 9 10 11 12 13 14 15 16 17 18 19
0
1
2
3
4
5
0
1
2
3
4
5
4 5 6 7 8 9 10 11 12 13 14 15 16 17 18 19
Black tea in
solvent vent mode
Following ACN
blank in solvent
vent mode
Black tea in
cold splitless mode
Following ACN blank
in cold splitless mode
1
2
3
4
8.6 8.7 8.8 8.9 9.0 9.1
Five sequential
blanks
Caffeine Caffeine
Clean blank
Same
scale
×108
×108
×108
Same
scale
×108
×108
Acquisition time (min)
Counts Counts
Counts Counts
Figure 9. Injection optimization for analyzing black tea: cold splitless (on the right) reduces carryover of caffeine compared to solvent vent mode (on the left).
87
13
HES 2.0: Novel electron ionization (EI) source technology
Equipped with the novel HES 2.0 EI source, the 7010D
demonstrated sensitivity that allows for ultra-trace level
detection when analyzing pesticides. The new HES 2.0 ion
source is equipped with a novel dipolar RF lens that redirects
the carrier gas ions and, as a result, enables improved system
robustness and unparalleled analytical sensitivity.
Figure 10 shows MRM chromatograms for selected
pesticides at 0.01 ppb in black tea extract. The overlaid
chromatograms show repeatability over seven replicate
injections, and the response RSD% as a measure of precision.
Appendix Table 1 shows the LOQs for all analyzed pesticides.
LOQs as low as 0.01 ppb were observed for 34% of the
targets, at or below 0.1 ppb for 74% of compounds, and below
2 ppb for 96%. The number of compounds expressed in
percent, with their respective LOQs, are plotted in Figure 11.
Figure 10. MRM chromatograms with seven replicate injections for the selected pesticides at the LOQ of 0.01 ppb in black tea extract and their calibration curves.
Bromophos-ethyl
Concentration (ng/mL)
0 200 400 600 800 1,000
Concentration (ng/mL)
0 200 400 600 800 1,000
Concentration (ng/mL)
0 200 400 600 800 1,000
Concentration (ng/mL)
0 200 400 600 800 1,000
Concentration (ng/mL)
0 200 400 600 800 1,000
-0.2
0
0.2
0.4
0.6
0.8
1.0
1.2
1.4
1.6
1.8
2.0
2.2
2.4 y = 23,817.677338x +
874.434909
R2
= 0.99951780
R = 0.99991699
RSE = 9.1
0.2
0.4
0.6
0.8
1.0
1.2
1.4
1.6
1.8
2.0
2.2
2.4
11.3 11.4 11.5 11.6
Chlorfenson
RSD = 9%
0.01 ppb
N = 7
3.5
4.0
4.5
5.0
5.5
6.0
6.5
7.0
7.5
8.0
8.5
10.9 11.0 11.1 11.2 11.3
Bromophos ethyl
RSD = 8%
0.01 ppb
N = 7
0.01 to 1,000 ppb 0.01 to 1,000 ppb
0.01 to 1,000 ppb 0.01 to 1,000 ppb
0.01 to 1,000 ppb
Chlorfenson
-0.1
0
0.1
0.2
0.3
0.4
0.5
0.6
0.7
0.8
0.9
1.0
1.1 y = 103,879.642468x +
3,894.601308
R2
= 0.99936894
R = 0.99990218
RSE = 10.2
DDE-o,p'
0
1
2
3
4
5
6
7
8
y = 83,323.889419x +
6,145.523095
R2
= 0.99949459
R = 0.99992324
RSE = 6.4
DDE-p,p'
0
1
2
3
4
5
6 y = 62,964.732203x +
6,196.477029
R2
= 0.99949744
R = 0.99993069
RSE = 6.8
0.4
0.6
0.8
1.0
1.2
1.4
1.6
1.8
2.0
2.2
2.4
2.6
2.8
3.0
11.0 11.2 11.4 11.6 11.8
DDE -o,p'
RSD = 5%
0.01 ppb
DDE -p,p'
RSD = 6%
0.01 ppb
N = 7
Chloropropylate
0
1
2
3
4
5
6
7
y = 72,085.499690x + 3,773.703370
R2
= 0.99957751
R = 0.99991508
RSE = 7.2
0.3
0.4
0.5
0.6
0.7
0.8
0.9
1.0
1.1
1.2
1.3
1.4
1.5
1.6
1.7
1.8
12.05 12.15 12.25 12.35
Chloropropylate
RSD = 12%
0.01 ppb
N = 7
×102
×103
×103
×107
×107
×107
×107 ×103 ×108
Acquisition time (min) Acquisition time (min)
Acquisition time (min)
Acquisition time (min)
Counts
Counts
Counts Counts
Responses Responses
Responses
Responses
Responses
88
14
Figure 11. Percentage of compounds with their respective LOQ levels (in
ppb) in black tea extract.
0%
20%
40%
60%
80%
100%
120%
0.01 0.05 0.1 0.5 1 2 10
Percent of compounds (out of 246)
with LOQ at or belo
w
LOQ (in ppb)
Figure 10 also shows the matrix-matched calibration
performance in black tea extract with excellent linearity
maintained over five orders of magnitude, ranging from 0.01
to 1,000 ppb. All calibration curves were inspected, and if
needed, were trimmed to comply with SANTE 11312/2021
guidelines.2
Appendix Table 1 provides information on
calibration ranges and quality of calibration fit for all
compounds. The R2
correlation coefficient for all targets
was > 0.99. The RSE was used as an additional criterion for
demonstrating the calibration curve quality. The RSE provides
an improved criterion for evaluation of calibration curves,
as it is consistent for evaluation of all curve fitting types.9
In
this work, the calibration curves for all compounds had RSE
values below 20.
For the compounds for which quadratic calibration fit was
used, a linear calibration curve fit can be used instead by
narrowing the calibration range. For example, oxyfluorfen
could be calibrated over five orders of magnitude, from 0.01
to 1,000 ppb using quadratic calibration fit with R2
= 0.9995
and RSE = 14. Alternatively, a linear calibration fit could be
applied over a calibration range of 0.01 to 500 ppb with
R2
= 0.9960 and RSE = 26. The selection of the calibration
curve fit was guided by the lower RSE value.
Some pesticides are known to present a particular challenge
for analysis. As stated in the EURL Analytical Observation
Report10, captan and folpet are analytically among the most
challenging pesticides due to their nonamenability to LC/TQ,
and their tendency to degrade both in solution as well as in
the GC inlet. Figure 12 demonstrates that captan and folpet
could be quantitated with great precision at LOQs as low as
2 and 0.5 ppb, respectively. Freshly diluted standard, acidified
sample extracts, and optimized injection conditions with
cold splitless injection were key to achieving high recoveries
and precision in analyzing captan and folpet. Deltamethrin, a
synthetic pyrethroid, elutes at the end of the chromatographic
run and is also known to be difficult for GC/MS analysis.11
As shown in Figure 12, deltamethrin could be reliably
quantitated down to 0.5 ppb using the developed method.
Other compounds shown in Figure 12 include organochlorine
pesticides, aldrin, dieldrin, and endrin, and the two most
widely used multipurpose pyrethroids, cypermethrin and
cyfluthrin, quantitated with great precision and excellent
linearity over a wide dynamic range.
89
15
Figure 12. MRM chromatograms with eight replicate injections for the selected challenging pesticides. Included are LOQ levels and their calibration curves.
0.3
0.4
0.5
0.6
0.7
0.8
0.9
1
1.1
1.2
1.3
1.4
1.5
1.6
1.7
1.8
1.9
9.8 9.9 10.0
Aldrin
RSD = 8%
0.5 ppb
N = 8
Aldrin
0
1
2
3
4
5
6
7 y = 6,985.401438x + 203.727553
R2
= 0.99952950
R = 0.99993060
RSE = 8.8
0.4
0.5
0.6
0.7
0.8
0.9
1.0
10.6 10.7 10.8 10.9 11.0
Folpet
RSD = 7%
0.5 ppb
Folpet
0 50 100 150 200
0
1
2
3
4
5
6 y = 2,692.336742x +
830.709066
R2
= 0.99889601
R = 0.99995851
RSE = 16.4
0.5 to 200 ppb
1 to 500 ppb
0.05 to 1,000 ppb
0.5 to 1,000 ppb
0.1 to 500 ppb 0.1 to 1,000 ppb
1.0
1.2
1.4
1.6
1.8
2.0
2.2
2.4
2.6
2.8
3.0
11.6 11.8 12.0 12.2
Endrin
RSD = 9%
0.5 ppb
Dieldrin
RSD = 12%
0.5 ppb
0.5
0.6
0.7
0.8
0.9
1.0
1.1
1.2
1.3
1.4
10.55 10.65 10.75 10.85 10.95
Captan
RSD = 10%
2 ppb
N = 8
N = 8
Endrin
0
1
2
3
4
5
6
7
y = 7,758.571223x +
792.052413
R2
= 0.99958608
R = 0.99993430
RSE = 5.5
Captan
-1
0
1
2
3
4
5
6
7 y = 1,198.868510x + 256.553484
R2
= 0.99734455
R = 0.99927622
RSE = 18.9
0.50
0.54
0.58
0.62
0.66
0.70
0.74
0.78
0.82
0.86
0.90
0.94
0.98
1.02
18.0 18.1 18.2 18.3 18.4
Deltamethrin
RSD = 10%
0.5 ppb
N = 8
Deltamethrin
0 100 200 300 400 500
-0.1
0
0.1
0.2
0.3
0.4
0.5
0.6
0.7
0.8
0.9
1.0
1.1
y = 1,873.813407x + 335.177249
R2
= 0.99627763
R = 0.99887520
RSE = 17.2
1.0
1.4
1.8
2.2
2.6
3.0
3.4
3.8
4.2
4.6
5.0
16.1 16.3 16.5 16.7
Cyfluthrins
RSD = 6%
1 ppb
Cypermethrins
RSD = 5%
1 ppb
N = 8
Cypermethrin I
0 200 400 600 800
-0.1
0
0.1
0.2
0.3
0.4
0.5
0.6
0.7
0.8
0.9
1.0
1.1
1.2
1.3 y = 12,792.103487x +
12,912.393135
R2
= 0.99849640
R = 0.99974393
RSE = 16.6
Concentration (ng/mL)
0 200 400 600 800
Concentration (ng/mL)
0 200 400 600 800
1,000
1,000
1,000
Concentration (ng/mL) Concentration (ng/mL)
Concentration (ng/mL)
0 100 200 300 400 500
Concentration (ng/mL)
×103
×103
×103
×105
×105
×106
×103
×103
×103
×106
×106
×107
Acquisition time (min)
Acquisition time (min)
Acquisition time (min) Acquisition time (min)
Acquisition time (min)
Acquisition time (min)
Counts Counts Counts
Counts Counts Counts
Responses Responses Responses
Responses Responses Responses
90
16
Recovery and precision
To validate the complete workflow solution and ensure that
enhanced matrix cleanup did not have a negative impact on
pesticide recovery, a study aimed at recovery and precision
evaluation was performed. Two concentrations were selected
for the study (10 and 50 ng/g) in dry black tea, resulting in 2
and 10 ppb in the final extract due to the 5x dilution factor.
Figure 13 shows target results at 10 and 50 ng/g in black
tea, demonstrating the acceptable recoveries achieved for
the majority of pesticides, even for the common problematic
pesticides such as planar and labile.
Longevity and maximized throughput with confidence
The ruggedness of the analysis was demonstrated by
analyzing a challenging black tea extract spiked with
pesticides at 2 ppb. The area of the analyte response was
monitored over 800 consecutive injections. Analyte response,
normalized by the internal standards (ISTD), remained
consistent over 800 injections that spanned over 400 hours
of continuous running with RSDs < 20% for 176 compounds.
Figure 14 shows the response for 60 compounds, normalized
by the ISTD and by the average response for each analyte.
0%
20%
40%
60%
80%
100%
120%
140%
160%
180%
Dichlorvos
Dichlorobenzonitrile, 2,6-
Biphenyl
Mevinphos, EChlormephos
Propham
Pebulate
Etridiazole
Nitrapyrin
cis-1,2,3,6-Tetrahydrophthalimide
Methacrifos
Chloroneb
Crimidine
2-Phenylphenol
Isoprocarb I
Pentachlorobenzene
Heptenophos
DEET
Chlorfenprop-methyl
Omethoate
Thionazin
Flonicamid
Propachlor
Ethoprophos
Cycloate
Chlorpropham
Ethalfluralin
DMSA
Trifluralin
Benfluralin
Monocrotophos
Dicrotofos
Sulfotep
Bromoxynil
Promecarb
Cadusafos
Phorate
BHC-alpha (benzene hexachloride)
Desmedipham
Hexachlorobenzene
Dichloran
Dimethoate
Pentachloroanisole
Propazine
BHC-beta
DMST (Tolylfluanid metabolite)
Propetamphos
Profluralin
BHC-gamma (Lindane, gamma HCH)
Cyanophos
Terbufos
Pentachloronitrobenzene
Fonofos
Diazinon
Pyrimethanil
Fluchloralin
Phosphamidon I
Dinitramine
Tefluthrin
Paraoxon-methyl
BHC-delta
Isazofos
Etrimfos
Triallate
Chlorothalonil
Iprobenfos
Formothion
Bromocyclen
Pentachloroaniline
Desmetryn
Dichlofenthion
Propanil
2,4,4'-Trichlorobiphenyl (BZ #28)
Malaoxon
Vinclozolin
Transfluthrin
Parathion-methyl
Chlorpyrifos-methyl
Cymiazole
Tolclofos-methyl
Alachlor
Fuberidazole
Heptachlor
Prometryn
Paraoxon
Ronnel
Prosulfocarb
Octachlorodipropyl ether
Pirimiphos-methyl
2,2',5,5'-Tetrachlorobiphenyl (BZ #52)
Fenitrothion
Methiocarb
Dipropetryn
Malathion
Ioxynil
Dichlofluanid
Parathion-d10
Metolachlor
Phorate sulfone
Aldrin
Anthraquinone
Chlorpyrifos
Parathion
Flufenacet
Nitrothal-isopropyl
DCPA (Dacthal, Chlorthal-dimethyl)
Isocarbophos
Chlorthion
Isobenzan
Trichloronat
Fenson
Bromophos
Pirimiphos-ethyl
Fosthiazate I
Isopropalin
Cyprodinil
Isofenphos-methyl
Isodrin
Pendimethalin
Terbufos sulfone
Chlozolinate
Heptachlor exo-epoxide
Esbiothrin
Bioallethrin
Chlordane-oxy
Tolylfluanid
Isofenphos
Mecarbam
Chlorfenvinphos
Heptachlor endo-epoxide
Fipronil
Captan
Quinalphos
Phenthoate
Dinobuton
Procymidone
Folpet
Chlorbenside
Methidathion
Bromophos-ethyl
Chlordane-trans
DDE-o,p'
2,2',4,5,5'-Pentachlorobiphenyl (BZ #101)
Tetrachlorvinphos
Chlordane-cis
Endosulfan I (alpha isomer)
Ditalimfos
Picoxystrobin
Flutriafol
Fenamiphos
Nonachlor, transChlorfenson
Iodofenphos
Prothiofos
Isoprothiolane
Flubenzimine
Profenofos
DDE-p,p'
Dieldrin
Oxyfluorfen
Myclobutanil
DDD-o,p'
Methoprotryne
Azaconazole
Dibromobenzophenone, 4,4'-
Isoxathion
Binapacryl
Nitrofen
Ethylan
Chlorfenapyr
Endrin
Carbophenothion-Methyl
Chloropropylate
2,3',4,4',5-Pentachlorobiphenyl (BZ #118)
Endosulfan II (beta isomer)
Fensulfothion
Flamprop-isopropyl
DDD-p,p'
Aclonifen
DDT-o,p'
Ethion
Chlorthiophos
Tetrasul
2,2',4,4',5,5'-Hexachlorobiphenyl (BZ #153)
Sulprofos
Triazophos
Famphur
Carbophenothion
Methoxychlor olefin
Cyanofenphos
Edifenphos
DDT-p,p'
Endosulfan sulfate
2,2',3,4,4',5'-Hexachlorobiphenyl (BZ #138)
Diclofop-methyl
Diflufenican
Propargite
Piperonyl butoxide
Captafol
Nitralin
Mefenpyr-diethyl
Benzoylprop-ethyl
Iprodione
Spiromesifen
Tetramethrin I
Pyridaphenthion
Endrin ketone
Dimoxystrobin
Phosmet
Bifenthrin
Bromopropylate
EPN
Picolinafen
Dicofol, p, p'-
Fenpropathrin
2,2',3,4,4',5,5'-Heptachlorobiphenyl (BZ #180)
Phenothrin I
Tetradifon
Furathiocarb
Phosalone
Azinphos-methyl
Leptophos
Cyhalothrin (Lambda)
Cyhalofop-butyl
Tralkoxydim
Mirex
Acrinathrin
Pyrazophos
Azinphos-ethyl
Cycloxydim (Focus)
Permethrin, (1R)-cisPermethrin, (1R)-transCoumaphos
Dioxathion
Butafenacil
Cyfluthrin I
Cypermethrin I
Halfenprox
Flucythrinate I
Ethofenprox
Silafluofen
Fenvalerate I
Fluvalinate-tau I
Deltamethrin
10 ppb in tea → 2 ppb in the extract 50 ppb in tea → 10 ppb in the extract
Figure 13. Pesticide recoveries in black tea at 10 and 50 ppb shown for all 244 pesticides.
Figure 14. Stability of the peak area for pesticides spiked at 2 ppb into black tea extract, normalized by the ISTD and the average response, over 800 consecutive
injections with the Agilent 8890 GC and 7010D GC/TQ systems.
100 200 300 400 500 600 700
BHC-alpha (benzene hexachloride) 11%
Coumaphos 15%
Fenitrothion 11%
Chlorpyrifos 11%
Ethalfluralin 10%
Esbiothrin 9%
Propazine 10%
Tefluthrin 10%
2,2',4,4',5,5'-Hexachlorobiphenyl (BZ #153) 9%
Aldrin 12%
Myclobutanil 11%
Chlordane-oxy 11%
BHC-beta 10%
DDD-o,p' 10%
Fenpropathrin 11%
Parathion 7%
DMSA 10%
Bioallethrin 9%
Diazinon 10%
Iprobenfos 9%
2,2',3,4,4',5'-Hexachlorobiphenyl (BZ #138) 9%
Dieldrin 10%
Nonachlor, trans- 10%
Chlorfenvinphos 11%
Bromophos 10%
DDT-p,p' 9%
Fonofos 12%
Fenson 9%
Trifluralin
DCPA (Dacthal, Chlorthal-dimethyl) 11%
Pyrimethanil 10%
2,2',5,5'-Tetrachlorobiphenyl (BZ #52) 10%
2,2',3,4,4',5,5'-Heptachlorobiphenyl (BZ #180) 9%
Endrin 15%
Chlorfenson 10%
Heptachlor endo-epoxide 10%
Prothiofos 10%
DDE-o,p' 8%
Piperonyl butoxide 11%
EPN 11%
Benfluralin 10%
Metolachlor 9%
Fluchloralin 8%
2,2',4,5,5'-Pentachlorobiphenyl (BZ #101) 9%
Bifenthrin 11%
Endrin ketone 11%
Chlordane-cis 10%
Heptachlor exo-epoxide 10%
Chlorthiophos 11%
DDE-p,p' 9%
Sulfotep 12%
Pentachloronitrobenzene 11%
Profluralin 9%
Pirimiphos-ethyl 10%
Dinitramine 11%
2,3',4,4',5-Pentachlorobiphenyl
(BZ #118) 9%
Chloropropylate 8%
Nitrofen 10%
Chlordane-trans 10%
Deltamethrin 25%
Column trim Replaced syringe
0%
20%
40%
60%
80%
100%
120%
140%
160%
180%
200%
91
17
The graph shows that analyte responses were stable and
within 80 to 120% throughout the entire study that lasted
over 17 days of continuous analysis. The RSDs for each of
the target responses are shown in the legend in Figure 14,
with most of them below 12%. The absolute responses in
terms of peak areas also remained consistent throughout the
longevity study. For example, the RSDs on the peak areas over
800 injections for early-eluting BHC-beta, mid-range eluting
fenson, and late-eluting coumaphos were 9%, 10%, and
16%, respectively.
The maintenance performed during the robustness testing
involved septum and liner replacement every 100 injections.
With the midcolumn backflush configuration and the use
of the temperature-programmed MMI inlet, inlet liner and
septum replacement could be performed in under four
minutes, providing a productivity boost to the workflow.
Two inches of the GC column head were trimmed after
500 injections. The use of the backflushing allowed for
a substantial increase in the number of injections before
column head maintenance was needed when analyzing a
complex black tea extract. Similar to GC inlet maintenance,
column trimming could be performed efficiently in a short
amount of time (5 to 10 minutes) and did not require MS
cool-down and venting due to the midcolumn configuration
coupled with the temperature-programmed MMI.
The autoinjector syringe was replaced after 600 injections
in the longevity study, as noted in Figure 14, with a total
of 1,000 injections made with the syringe. The decision
to replace the syringe was driven by the decreased
measurement precision resulting from a higher variability in
the target and ISTD responses. The replacement procedure
was performed as guided by the user manual for the 7693A
ALS.12 The precision of measurements was restored after
the syringe replacement. As a result, the graph in Figure 14
shows an increased response variability between 500 and
600 injections. This effect was particularly pronounced for
deltamethrin, which can present a challenge for achieving
good precision at low concentrations. Washing the syringe
needle support foot is an additional autoinjector maintenance
procedure to consider when analyzing challenging samples to
minimize carryover and ensure precision.
It is of note that there was no need to perform GC inlet or MS
source cleaning during the entire study, which spanned over
1,000 injections, including the calibration assessment and
precision and recovery studies.
The exceptional method ruggedness shown in this work was
achieved by:
– Following the key practices to successful pesticide
analysis outlined in this application note and in another
application note7
– Performing effective sample preparation and cleanup
– Using the state-of-the art GC and MS technology in the
8890 and 7010D GC/TQ systems
GC/TQ intelligence and new software functionality
The health and status of the GC/TQ system was continuously
monitored though the longevity study by using the Early
Maintenance Feedback functionality in MassHunter
Acquisition 13.0 for GC/MS. Figure 15A shows a screenshot
of the MS health status, featuring the electron multiplier
(EM) voltage at last tune, filament age, pump maintenance
schedule, and time since the source was cleaned. The
dashboard allows for daily tracking for essential maintenance
procedures and reminds the user to perform maintenance on
time. In addition to the dashboard view, the built-in intelligent
functionality in the 7010D GC/TQ system allows for plotting
tune-related parameters over time to track the EI source
health and performance. The plot for the EM voltage is shown
in Figure 15B. The routine tune check procedures, which could
be built into the sequence table via keywords, are helpful in
guiding when the EM gain curve needs to be updated. This
procedure allows for adjusting the EM voltage to maintain a
stable response while not altering tune parameters and ion
ratio, maintaining MS tune and method calibration validity.
92
18
A
B
Consider maintenance
EMV monitored over a five-month duration of experiment preparation and execution
Figure 15. The early maintenance dashboard for GC/TQ (A) and the EM voltage plot for the tune history (B) shown in Agilent MassHunter Acquisition 13.0
for GC/MS.
93
19
Conclusion
This application note presents a workflow solution for
analyzing pesticides in black tea with the new 7010D GC/TQ,
allowing for the quantitation of 246 pesticide residues at
trace levels with LOQs as low as 0.01 ppb for 34% of the
targets, at or below 0.1 ppb for 74% of compounds, and
below 2 ppb for 96%. Matrix-matched calibration allowed for
excellent accuracy over a wide dynamic range, spanning up
to five orders of magnitude over the 0.01 to 1,000 ppb range
in a complex black tea extract. Method ruggedness was
demonstrated through maintaining measurement accuracy
with good precision (RSDs < 20% for 176 compounds) for
black tea extract spiked at 2 ppb sequentially analyzed over
800 runs and spanning over 17 days of continuous analysis.
The key components for a robust workflow included a
combination of efficient sample preparation and cleanup, the
Agilent 8890 GC hardware, functionality, and GC supplies, the
novel EI source technology with HES 2.0, and lastly, the built-in
GC/TQ intelligence and new software functionality.
References
1. Mehri, A.; Taleb, R.; Elaridi, J.; Hassan, H. F. Analytical
Methods Used to Determine Pesticide Residues in Tea: A
Systematic Review. Appl. Food Res. 2022, 2(1), 100131.
2. Analytical Quality Control and Method Validation
Procedures for Pesticide Residues Analysis in Food and
Feed. SANTE 11312/2021, 2021.
3. Tolerances and Exemptions for Pesticide Chemical
Residues in Food. Title 40 U.S. Code of Federal
Regulations, US EPA.
4. Lozano, A.; Rajski, L.; Belmonte-Valles N.; Uclés, A.; Uclés,
S.; Mezcua, M.; Fernández-Alba, A. Pesticide Analysis in
Teas and Chamomile by Liquid Chromatography and Gas
Chromatography Tandem Mass Spectrometry Using a
Modified QuEChERS Method: Validation and Pilot Survey
in Real Samples. J. Chrom. A 2012, 1268, 109–122.
5. The Agilent MassHunter Pesticide and Environmental
Pollutants MRM Database (P&EP 4.0). G9250AA. https://
www.agilent.com/en/product/gas-chromatographymass-spectrometry-gc-ms/gc-ms-application-solutions/
pesticides-environmental-pollutants-4-0-mrm-database
6. Maštovská, K.; Lehotay, S. J.; Anastassiades, M.
Combination of Analyte Protectants to Overcome Matrix
Effects in Routine GC Analysis of Pesticide Residues in
Food Matrixes. Anal. Chem. 2005, 77, 8129–8137
7. Five Keys to Unlock Maximum Performance in the
Analysis of Over 200 Pesticides in Challenging Food
Matrices by GC/MS/MS. Agilent Technologies application
note 5994-4965EN, 2022.
8. Using the PSD for Backflushing on the Agilent 8890 GC
System. Agilent Technologies application note, publication
number 5994 0550EN, 2018.
9. Burrows, R. Parr, R. Evaluating the Goodness of
Instrument Calibration for Chromatography Procedures.
LCGC Supplements Special Issues 2020 11-01-20, 38(11),
35–38.
10. EURL-SRM – Analytical Observation Report.
Quantification of Residues of Folpet and Captan in
QuEChERS Extracts Version 3.1 (last update: 06.04.17).
11. Kim, L.; Baek, S.; Son, K.; Kim, E.; Noh, H. H.; Kim, D.;
Oh, M.; Moon, B.; Ro, J.-H. Optimization of a Simplified
and Effective Analytical Method of Pesticide Residues
in Mealworms (Tenebrio molitor Larvae) Combined with
GC–MS/MS and LC–MS/MS. Mol. 2020, 25(15), 3518.
12. Agilent 7693A Automatic Liquid Sampler. Installation,
Operation and Maintenance. Agilent Technologies, 2023.
94
20
Name RT Transition Calibration Range (ppb) CF CF R2
Relative Standard
Error
Methamidophos 4.520 141.0 & 64.0 0.1 – 1,000 Linear 0.9994 7.5
Dichlorvos 4.643 184.9 & 93.0 0.05 – 1,000 Linear 0.9988 11.2
Dichlorobenzonitrile, 2,6- 5.210 171.0 & 100.0 0.01 – 1,000 Linear 0.9990 10.4
Biphenyl 5.390 154.1 & 153.1 0.5 – 1,000 Quadratic 0.9991 11.8
Mevinphos, E- 5.578 127.0 & 94.9 0.5 – 1,000 Linear 0.9994 7.9
Acephate 5.679 136.0 & 94.0 5 – 1,000 Quadratic 0.9990 8.1
Chlormephos 5.687 153.9 & 121.1 0.5 – 1,000 Linear 0.9979 5.0
Propham 5.740 178.9 & 137.1 1 – 1,000 Linear 0.9986 9.6
Pebulate 5.774 128.0 & 57.1 0.5 – 1,000 Linear 0.9983 6.6
Etridiazole 5.798 213.1 & 185.0 0.1 – 500 Linear 0.9986 10.7
Nitrapyrin 5.804 194.0 & 158.0 0.05 – 1,000 Quadratic 0.9994 8.7
cis-1,2,3,6-Tetrahydrophthalimide 5.956 151.1 & 80.0 0.1 – 1,000 Linear 0.9994 6.1
Methacrifos 6.027 207.9 & 180.1 0.05 – 1,000 Quadratic 0.9996 11.4
Chloroneb 6.110 191.0 & 113.0 0.01 – 1,000 Linear 0.9995 8.2
Crimidine 6.212 170.9 & 142.1 0.1 – 1,000 Linear 0.9994 10.4
2-Phenylphenol 6.213 169.1 & 115.1 0.5 – 1,000 Linear 0.9995 5.6
Isoprocarb I 6.295 136.0 & 121.1 0.5 – 1,000 Linear 0.9994 9.3
Pentachlorobenzene 6.311 251.9 & 217.0 0.01 – 1,000 Linear 0.9984 11.4
Heptenophos 6.585 124.0 & 89.0 0.01 – 500 Linear 0.9990 12.5
DEET 6.600 191.0 & 190.0 0.5 – 1,000 Linear 0.9981 11.5
Chlorfenprop-methyl 6.696 165.0 & 102.0 0.01 – 1,000 Linear 0.9989 14.0
Omethoate 6.773 110.0 & 47.0 0.1 – 1,000 Quadratic 0.9997 8.8
Thionazin 6.781 143.0 & 79.0 0.1 – 1,000 Quadratic 0.9998 9.5
Flonicamid 6.859 173.9 & 68.9 0.01 – 1,000 Linear 0.9994 11.5
Propachlor 6.865 176.1 & 57.1 0.05 – 1,000 Quadratic 0.9997 8.5
Ethoprophos 6.996 157.9 & 97.0 0.1 – 1,000 Quadratic 0.9997 8.2
Cycloate 7.017 154.1 & 83.1 0.05 – 1,000 Linear 0.9991 16.2
Chlorpropham 7.080 171.0 & 127.1 0.05 – 500 Linear 0.9993 13.7
Ethalfluralin 7.109 275.9 & 202.1 0.05 – 1,000 Quadratic 0.9997 11.4
DMSA 7.169 200.0 & 108.0 2 – 1,000 Quadratic 0.9963 17.5
Trifluralin 7.217 306.1 & 264.0 0.05 – 1,000 Quadratic 0.9997 11.8
Benfluralin 7.251 292.0 & 264.0 0.1 – 1,000 Quadratic 0.9996 12.0
Monocrotophos 7.258 192.0 & 127.0 0.1 – 1,000 Quadratic 0.9998 7.4
Dicrotofos 7.264 193.0 & 127.1 0.5 – 1,000 Quadratic 0.9998 10.1
Sulfotep 7.349 321.8 & 201.9 0.05 – 1,000 Quadratic 0.9997 10.4
Bromoxynil 7.395 276.8 & 88.0 0.05 – 1,000 Quadratic 0.9997 7.1
Promecarb 7.399 135.1 & 115.1 2 – 1,000 Linear 0.9967 16.1
Cadusafos 7.405 158.8 & 97.0 0.01 – 1,000 Quadratic 0.9997 13.1
Phorate 7.475 121.0 & 47.0 0.5 – 1,000 Linear 0.9983 11.1
BHC-alpha (Benzene Hexachloride) 7.609 218.9 & 183.0 0.01 – 1,000 Quadratic 0.9998 9.9
Desmedipham 7.690 181.0 & 122.0 2 – 1,000 Linear 0.9985 11.8
Hexachlorobenzene 7.741 283.8 & 213.9 0.01 – 1,000 Quadratic 0.9996 10.7
Dichloran 7.771 160.1 & 124.1 0.01 – 1,000 Linear 0.9996 6.7
Dimethoate 7.781 87.0 & 46.0 0.01 – 1,000 Linear 0.9997 11.8
Pentachloroanisole 7.797 279.9 & 236.8 0.05 – 1,000 Linear 0.9993 8.0
Appendix Table 1. Calibration performance for 246 pesticides in black tea using the Agilent 7010D GC/TQ equipped with the Agilent High-Efficiency Source (HES)
2.0.
95
21
Name RT Transition Calibration Range (ppb) CF CF R2
Relative Standard
Error
Propazine 7.933 229.1 & 58.1 0.01 – 1,000 Linear 0.9988 13.5
BHC-beta 8.010 218.9 & 183.1 0.01 – 1,000 Linear 0.9994 12.2
DMST (Tolylfluanid Metabolite) 8.032 214.0 & 106.0 2 – 1,000 Quadratic 0.9954 17.7
Propetamphos 8.079 138.0 & 64.0 0.1 – 1,000 Linear 0.9988 6.9
Profluralin 8.087 318.1 & 199.1 0.05 – 1,000 Quadratic 0.9997 12.7
BHC-gamma (Lindane,
Gamma HCH) 8.119 216.9 & 181.0 0.01 – 1,000 Linear 0.9981 15.6
Cyanophos 8.135 242.9 & 109.0 0.05 – 1,000 Linear 0.9993 12.8
Terbufos 8.137 230.9 & 129.0 0.1 – 1,000 Linear 0.9994 12.5
Pentachloronitrobenzene 8.195 141.9 & 106.9 0.01 – 1,000 Quadratic 0.9996 9.4
Fonofos 8.223 246.1 & 109.0 0.01 – 500 Linear 0.9981 11.9
Diazinon 8.264 137.1 & 84.0 0.05 – 1,000 Quadratic 0.9997 17.0
Pyrimethanil 8.269 198.0 & 118.1 0.01 – 500 Linear 0.9990 12.6
Fluchloralin 8.299 325.8 & 62.9 0.05 – 500 Quadratic 0.9995 13.5
Phosphamidon I 8.339 127.0 & 95.0 0.5 – 500 Linear 0.9981 17.4
Dinitramine 8.382 260.7 & 241.0 0.05 – 1,000 Quadratic 0.9997 11.6
Tefluthrin 8.400 177.1 & 127.1 0.01 – 1,000 Linear 0.9986 16.0
Paraoxon-methyl 8.411 229.9 & 106.1 0.05 – 500 Linear 0.9947 18.3
BHC-delta 8.489 219.0 & 183.1 0.5 – 1,000 Quadratic 0.9998 16.5
Isazofos 8.504 256.9 & 162.0 0.01 – 1,000 Quadratic 0.9997 13.8
Etrimfos 8.523 292.0 & 153.1 0.01 – 500 Linear 0.9985 16.7
Triallate 8.540 268.0 & 184.1 0.05 – 1,000 Linear 0.9995 10.8
Chlorothalonil 8.568 265.9 & 168.0 0.1 – 500 Quadratic 0.9971 10.7
Iprobenfos 8.673 203.9 & 91.0 0.01 – 1,000 Linear 0.9986 13.9
Formothion 8.763 124.9 & 47.0 0.1 – 1,000 Quadratic 0.9997 16.0
Bromocyclen 8.764 271.8 & 236.9 0.1 – 1,000 Linear 0.9996 8.6
Pentachloroaniline 8.897 158.0 & 123.0 0.5 – 1,000 Linear 0.9986 14.7
Desmetryn 8.916 213.0 & 58.1 0.05 – 1,000 Linear 0.9972 11.7
Dichlofenthion 8.961 279.0 & 223.0 0.01 – 500 Linear 0.9975 11.4
Propanil 8.980 161.0 & 99.0 0.01 – 1,000 Linear 0.9983 16.8
2,4,4'-Trichlorobiphenyl (BZ #28) 9.030 256.0 & 186.0 0.05 – 1,000 Linear 0.9981 11.7
Malaoxon 9.103 126.9 & 99.0 2 – 1,000 Quadratic 0.9989 15.0
Vinclozolin 9.128 187.0 & 124.0 0.05 – 1,000 Linear 0.9962 15.8
Transfluthrin 9.129 163.1 & 143.1 0.1 – 1,000 Linear 0.9972 14.3
Parathion-methyl 9.151 262.9 & 109.0 0.5 – 500 Quadratic 0.9991 18.2
Chlorpyrifos-methyl 9.151 288.0 & 93.0 0.05 – 1,000 Quadratic 0.9995 12.0
Cymiazole 9.213 218.0 & 144.1 2 – 1,000 Linear 0.9986 12.3
Tolclofos-methyl 9.242 267.0 & 93.0 0.5 – 1,000 Linear 0.9987 12.8
Alachlor 9.280 237.0 & 160.1 1 – 1,000 Linear 0.9969 12.2
Fuberidazole 9.306 184.0 & 156.2 5 – 500 Linear 0.9921 19.5
Heptachlor 9.330 271.7 & 236.9 0.1 – 200 Linear 0.9990 15.4
Prometryn 9.339 241.0 & 58.2 5 – 200 Quadratic 0.9967 19.4
Paraoxon 9.383 148.9 & 119.0 50 – 1,000 Quadratic 0.9982 11.3
Ronnel 9.411 286.9 & 272.0 1 – 1,000 Linear 0.9989 11.7
Prosulfocarb 9.424 251.0 & 128.2 0.1 – 1,000 Quadratic 0.9995 14.9
Octachlorodipropyl Ether 9.431 129.9 & 94.9 0.5 – 1,000 Quadratic 0.9996 10.0
Pirimiphos-methyl 9.610 290.0 & 125.0 0.01 – 1,000 Quadratic 0.9996 9.3
96
22
Name RT Transition Calibration Range (ppb) CF CF R2
Relative Standard
Error
2,2',5,5'-Tetrachlorobiphenyl
(BZ #52) 9.617 289.9 & 219.9 0.01 – 1,000 Linear 0.9997 11.9
Fenitrothion 9.622 125.1 & 47.0 0.01 – 1,000 Linear 0.9994 11.3
Methiocarb 9.628 168.0 & 109.1 2 – 1,000 Quadratic 0.9998 11.3
Dipropetryn 9.748 255.1 & 222.1 0.01 – 500 Linear 0.9982 13.5
Malathion 9.759 172.9 & 99.0 0.01 – 1,000 Quadratic 0.9997 12.1
Ioxynil 9.780 370.8 & 117.0 0.05 – 1,000 Linear 0.9982 12.2
Dichlofluanid 9.785 167.0 & 97.0 1 – 500 Quadratic 0.9991 13.8
Metolachlor 9.913 238.0 & 162.2 0.01 – 1,000 Linear 0.9993 12.5
Phorate Sulfone 9.914 199.0 & 97.0 0.1 – 500 Linear 0.9975 11.7
Aldrin 9.932 254.9 & 220.0 0.05 – 1,000 Linear 0.9995 8.8
Anthraquinone 9.941 208.0 & 152.2 0.05 – 1,000 Linear 0.9990 19.1
Chlorpyrifos 9.968 313.8 & 257.8 0.05 – 1,000 Linear 0.9994 11.7
Parathion 9.983 291.0 & 109.0 0.01 – 1,000 Linear 0.9985 15.2
Flufenacet 10.004 151.0 & 95.0 0.5 – 1,000 Linear 0.9994 11.9
Nitrothal-isopropyl 10.057 254.0 & 212.0 0.5 – 500 Linear 0.9967 16.3
DCPA (Dacthal, Chlorthal-dimethyl) 10.068 298.9 & 221.0 0.01 – 1,000 Linear 0.9993 14.1
Isocarbophos 10.114 136.0 & 69.0 0.5 – 1,000 Linear 0.9985 13.1
Chlorthion 10.156 125.1 & 47.1 0.05 – 1,000 Quadratic 0.9995 14.7
Isobenzan 10.186 274.7 & 240.0 0.1 – 1,000 Linear 0.9992 11.2
Trichloronat 10.199 296.8 & 268.9 0.01 – 1,000 Linear 0.9987 14.5
Fenson 10.210 141.0 & 77.1 0.01 – 1,000 Linear 0.9996 6.7
Bromophos 10.294 330.9 & 315.9 0.01 – 1,000 Linear 0.9986 15.8
Pirimiphos-ethyl 10.294 318.1 & 166.1 0.05 – 1,000 Linear 0.9977 14.8
Fosthiazate I 10.299 195.0 & 103.0 0.05 – 1,000 Quadratic 0.9997 12.1
Isopropalin 10.350 280.1 & 238.1 0.01 – 500 Linear 0.9975 16.4
Cyprodinil 10.413 225.2 & 224.3 0.05 – 1,000 Linear 0.9990 14.4
Isofenphos-methyl 10.420 199.0 & 121.0 0.01 – 1,000 Quadratic 0.9995 9.9
Isodrin 10.442 193.0 & 123.0 0.05 – 1,000 Linear 0.9985 10.9
Pendimethalin 10.522 251.8 & 162.2 0.05 – 1,000 Quadratic 0.9995 11.5
Terbufos Sulfone 10.573 264.0 & 199.0 0.05 – 1,000 Quadratic 0.9996 8.7
Chlozolinate 10.586 186.0 & 109.0 0.1 – 1,000 Quadratic 0.9995 8.6
Heptachlor Exo-epoxide 10.616 354.8 & 264.9 0.01 – 1,000 Linear 0.9993 15.3
Esbiothrin 10.622 123.0 & 93.0 2 – 1,000 Linear 0.9975 15.2
Bioallethrin 10.629 123.0 & 81.0 2 – 1,000 Linear 0.9924 12.2
Chlordane-oxy 10.629 114.9 & 51.1 0.5 – 1,000 Linear 0.9996 3.8
Tolylfluanid 10.639 238.0 & 137.0 0.05 – 1,000 Quadratic 0.9996 14.6
Isofenphos 10.674 212.9 & 121.1 0.01 – 1,000 Quadratic 0.9994 10.7
Mecarbam 10.675 130.9 & 86.0 0.5 – 1,000 Quadratic 0.9992 13.0
Chlorfenvinphos 10.679 266.9 & 159.0 0.01 – 200 Linear 0.9986 11.0
Heptachlor Endo-epoxide 10.683 135.0 & 99.0 0.5 – 1,000 Linear 0.9993 6.6
Fipronil 10.698 366.8 & 212.8 0.05 – 1,000 Quadratic 0.9997 8.8
Captan 10.738 149.0 & 70.0 1 – 500 Linear 0.9973 18.9
Quinalphos 10.738 298.0 & 156.0 0.01 – 500 Quadratic 0.9992 17.6
Phenthoate 10.741 274.0 & 125.0 0.01 – 1,000 Quadratic 0.9988 8.1
Dinobuton 10.743 211.0 & 163.0 0.5 – 1,000 Quadratic 0.9999 9.0
Procymidone 10.850 282.8 & 96.0 0.01 – 1,000 Linear 0.9996 8.3
97
23
Name RT Transition Calibration Range (ppb) CF CF R2
Relative Standard
Error
Folpet 10.851 259.8 & 130.1 0.5 – 200 Linear 0.9989 16.4
Chlorbenside 10.904 125.0 & 89.0 0.01 – 1,000 Linear 0.9993 8.2
Methidathion 11.007 125.0 & 47.0 0.5 – 1,000 Linear 0.9993 16.2
Bromophos-ethyl 11.022 358.7 & 302.8 0.01 – 1,000 Linear 0.9995 9.1
Chlordane-trans 11.024 271.7 & 236.9 0.05 – 1,000 Linear 0.9995 8.4
DDE-o,p' 11.073 246.0 & 176.2 0.01 – 1,000 Linear 0.9995 6.4
2,2',4,5,5'-Pentachlorobiphenyl
(BZ #101) 11.111 325.9 & 255.9 0.01 – 1,000 Linear 0.9995 7.7
Tetrachlorvinphos 11.166 329.0 & 108.9 0.01 – 1,000 Linear 0.9972 16.9
Chlordane-cis 11.288 372.8 & 265.9 0.01 – 1,000 Linear 0.9994 15.4
Endosulfan I (Alpha Isomer) 11.290 194.9 & 160.0 0.1 – 1,000 Linear 0.9993 14.8
Ditalimfos 11.299 242.9 & 148.1 0.05 – 500 Linear 0.9977 14.8
Picoxystrobin 11.307 145.0 & 102.1 0.05 – 1,000 Linear 0.9994 14.1
Flutriafol 11.335 123.1 & 75.1 0.05 – 1,000 Linear 0.9995 11.9
Fenamiphos 11.360 303.0 & 154.0 0.1 – 500 Linear 0.9959 17.1
Nonachlor, trans- 11.369 406.8 & 299.8 0.05 – 1,000 Linear 0.9993 11.9
Chlorfenson 11.374 175.0 & 111.0 0.01 – 1,000 Linear 0.9994 10.2
Iodofenphos 11.466 376.8 & 361.8 0.05 – 1,000 Quadratic 0.9998 12.4
Prothiofos 11.488 308.9 & 238.9 0.05 – 500 Linear 0.9975 16.8
Isoprothiolane 11.498 162.1 & 85.0 0.01 – 1,000 Linear 0.9986 13.4
Flubenzimine 11.538 186.0 & 69.0 2 – 500 Quadratic 0.9974 10.5
Profenofos 11.544 207.9 & 63.0 0.1 – 200 Linear 0.9984 12.4
DDE-p,p' 11.613 246.1 & 176.2 0.01 – 1,000 Linear 0.9995 6.8
Dieldrin 11.713 262.9 & 193.0 0.5 – 1,000 Linear 0.9990 13.8
Oxyfluorfen 11.721 252.0 & 146.0 0.01 – 1,000 Quadratic 0.9995 14.0
Myclobutanil 11.750 179.0 & 125.1 0.01 – 1,000 Quadratic 0.9995 12.6
DDD-o,p' 11.783 235.0 & 165.1 0.01 – 1,000 Linear 0.9993 12.7
Methoprotryne 11.788 256.0 & 212.1 0.01 – 1,000 Quadratic 0.9996 12.6
Azaconazole 11.865 217.0 & 173.1 0.01 – 1,000 Linear 0.9994 11.4
Dibromobenzophenone, 4,4'- 11.913 340.0 & 183.0 0.05 – 1,000 Quadratic 0.9997 7.6
Isoxathion 11.941 313.0 & 177.0 0.05 – 1,000 Quadratic 0.9993 14.9
Binapacryl 12.003 100.0 & 82.0 5 – 1,000 Quadratic 0.9988 18.9
Nitrofen 12.011 282.9 & 253.0 0.01 – 500 Linear 0.9963 14.5
Ethylan 12.041 223.1 & 193.1 0.05 – 500 Linear 0.9976 14.7
Chlorfenapyr 12.051 328.0 & 247.0 0.5 – 1,000 Linear 0.9983 14.8
Endrin 12.108 262.8 & 193.0 0.5 – 1,000 Linear 0.9996 5.5
Carbophenothion-methyl 12.167 125.0 & 47.0 0.1 – 1,000 Linear 0.9986 19.2
Chloropropylate 12.187 139.0 & 75.0 0.01 – 1,000 Linear 0.9996 7.2
2,3',4,4',5-Pentachlorobiphenyl
(BZ #118) 12.222 325.9 & 255.9 0.01 – 1,000 Linear 0.9996 7.6
Endosulfan II (Beta Isomer) 12.274 206.9 & 172.0 0.1 – 1,000 Quadratic 0.9989 13.0
Fensulfothion 12.284 293.0 & 97.0 0.01 – 1,000 Linear 0.9978 14.8
Flamprop-isopropyl 12.305 276.0 & 105.1 0.05 – 1,000 Linear 0.9991 10.4
DDD-p,p' 12.369 237.0 & 165.1 0.01 – 1,000 Linear 0.9993 13.7
Aclonifen 12.397 264.1 & 194.2 0.1 – 1,000 Quadratic 0.9995 10.3
DDT-o,p' 12.430 235.0 & 199.1 0.01 – 1,000 Linear 0.9974 19.4
Ethion 12.431 231.0 & 129.0 0.01 – 1,000 Linear 0.9962 19.9
98
24
Name RT Transition Calibration Range (ppb) CF CF R2
Relative Standard
Error
Chlorthiophos 12.484 268.9 & 205.1 0.05 – 1,000 Linear 0.9983 13.9
Tetrasul 12.572 321.7 & 252.0 0.01 – 1,000 Linear 0.9987 11.5
2,2',4,4',5,5'-Hexachlorobiphenyl
(BZ #153) 12.610 359.9 & 289.9 0.01 – 1,000 Linear 0.9992 10.9
Sulprofos 12.650 322.0 & 156.0 0.01 – 500 Linear 0.9975 12.5
Triazophos 12.662 161.2 & 134.2 1 – 500 Quadratic 0.9995 15.0
Famphur 12.810 218.0 & 109.0 2 – 1,000 Linear 0.9982 14.6
Carbophenothion 12.826 342.0 & 157.0 0.05 – 500 Linear 0.9974 14.5
Methoxychlor Olefin 12.837 308.0 & 238.0 0.01 – 1,000 Linear 0.9984 13.5
Cyanofenphos 12.906 169.0 & 77.1 0.1 – 1,000 Linear 0.9988 10.7
Edifenphos 12.940 309.9 & 172.9 0.5 – 1,000 Quadratic 0.9998 13.3
DDT-p,p' 13.027 235.0 & 165.2 0.01 – 1,000 Linear 0.9976 19.9
Endosulfan Sulfate 13.032 271.9 & 237.0 0.5 – 1,000 Quadratic 0.9997 18.2
2,2',3,4,4',5'-Hexachlorobiphenyl
(BZ #138) 13.118 359.9 & 289.9 0.01 – 1,000 Linear 0.9996 6.5
Diclofop-methyl 13.284 339.9 & 252.9 0.01 – 500 Linear 0.9973 13.1
Diflufenican 13.310 266.0 & 246.1 0.01 – 1,000 Linear 0.9971 18.7
Propargite 13.327 231.0 & 135.0 0.5 – 1,000 Linear 0.9993 13.7
Piperonyl Butoxide 13.380 176.1 & 103.1 0.1 – 1,000 Quadratic 0.9995 9.0
Captafol 13.440 310.8 & 78.8 10 – 1,000 Quadratic 0.9987 19.3
Nitralin 13.551 315.9 & 274.0 0.1 – 500 Quadratic 0.9993 13.9
Mefenpyr-diethyl 13.608 253.0 & 189.0 0.01 – 500 Quadratic 0.9989 15.8
Benzoylprop-ethyl 13.699 292.0 & 105.0 0.1 – 1,000 Linear 0.9991 11.8
Iprodione 13.721 313.8 & 55.9 0.1 – 500 Linear 0.9984 12.4
Spiromesifen 13.722 272.0 & 254.2 0.1 – 500 Quadratic 0.9994 15.7
Tetramethrin I 13.814 164.0 & 77.1 5 – 1,000 Quadratic 0.9990 17.1
Pyridaphenthion 13.822 340.0 & 199.0 0.05 – 1,000 Quadratic 0.9996 12.9
Endrin Ketone 13.876 316.9 & 101.0 0.01 – 500 Quadratic 0.9993 13.6
Dimoxystrobin 13.880 205.0 & 58.0 0.1 – 1,000 Quadratic 0.9996 8.4
Phosmet 13.917 160.0 & 77.1 2 – 1,000 Quadratic 0.9993 10.6
Bifenthrin 13.922 181.2 & 165.2 0.1 – 500 Quadratic 0.9990 13.8
Bromopropylate 13.928 338.8 & 182.9 0.05 – 1,000 Quadratic 0.9993 10.7
EPN 13.935 169.0 & 77.1 0.05 – 1,000 Quadratic 0.9991 13.3
Picolinafen 13.958 376.0 & 238.1 0.01 – 200 Linear 0.9978 17.1
Bifenazate 13.975 168.1 & 61.9 10 – 1,000 Quadratic 0.9990 6.6
Dicofol, p, p'- 13.976 183.9 & 141.2 1 – 1,000 Linear 0.9972 18.8
Fenpropathrin 14.056 265.0 & 89.0 0.01 – 1,000 Quadratic 0.9996 13.5
2,2',3,4,4',5,5'-Heptachlorobiphenyl
(BZ #180) 14.299 393.8 & 323.8 0.01 – 1,000 Linear 0.9992 12.2
Phenothrin I 14.399 122.9 & 81.1 0.1 – 1,000 Linear 0.9987 11.5
Tetradifon 14.424 158.9 & 111.0 0.01 – 1,000 Linear 0.9992 7.4
Furathiocarb 14.437 163.1 & 135.1 2 – 1,000 Linear 0.9992 6.1
Phosalone 14.590 182.0 & 75.0 0.05 – 500 Linear 0.9958 19.2
Azinphos-methyl 14.626 160.0 & 77.0 2 – 1,000 Quadratic 0.9993 11.4
Leptophos 14.638 171.0 & 51.0 0.05 – 1,000 Quadratic 0.9995 12.4
Cyhalothrin (Lambda) 14.698 181.1 & 152.1 5 – 1,000 Quadratic 0.9979 12.9
Cyhalofop-butyl 14.703 357.1 & 229.1 0.01 – 500 Linear 0.9958 16.1
99
www.agilent.com
DE28615044
This information is subject to change without notice.
© Agilent Technologies, Inc. 2024
Printed in the USA, May 16, 2024
5994-7436EN
Name RT Transition Calibration Range (ppb) CF CF R2
Relative Standard
Error
Tralkoxydim 14.830 137.0 & 57.0 0.05 – 1,000 Linear 0.9990 7.9
Mirex 14.865 271.8 & 236.8 0.01 – 1,000 Linear 0.9994 13.5
Acrinathrin 15.045 247.0 & 68.0 1 – 1,000 Quadratic 0.9996 12.8
Pyrazophos 15.144 221.0 & 149.0 0.01 – 1,000 Quadratic 0.9994 14.1
Azinphos-ethyl 15.228 160.0 & 77.1 0.5 – 1,000 Quadratic 0.9997 12.4
Cycloxydim (Focus) 15.500 178.0 & 80.9 0.1 – 1,000 Quadratic 0.9997 8.0
Permethrin, (1R)-cis- 15.622 163.0 & 91.0 2 – 1,000 Quadratic 0.9978 18.4
Permethrin, (1R)-trans- 15.744 163.0 & 127.0 0.01 – 1,000 Linear 0.9990 12.0
Coumaphos 15.880 361.9 & 109.0 0.05 – 500 Linear 0.9972 16.0
Dioxathion 15.963 271.0 & 96.9 0.1 – 500 Linear 0.9969 19.8
Butafenacil 15.988 331.0 & 180.0 0.01 – 500 Linear 0.9973 14.4
Cyfluthrin I 16.202 163.0 & 127.0 0.5 – 1,000 Linear 0.9980 15.8
Cypermethrin I 16.510 163.0 & 127.0 0.1 – 1,000 Linear 0.9985 16.6
Halfenprox 16.565 262.9 & 169.0 0.05 – 1,000 Quadratic 0.9994 11.9
Flucythrinate I 16.725 156.9 & 107.1 0.01 – 1,000 Linear 0.9992 13.7
Ethofenprox 16.798 163.0 & 107.1 0.1 – 1,000 Linear 0.9989 14.3
Silafluofen 16.944 286.0 & 207.0 0.1 – 1,000 Quadratic 0.9995 9.7
Fenvalerate I 17.428 167.0 & 125.1 0.05 – 1,000 Linear 0.9988 12.6
Fluvalinate-tau I 17.601 250.0 & 200.0 0.1 – 1,000 Quadratic 0.9996 15.2
Deltamethrin 18.152 252.9 & 174.0 0.1 – 500 Linear 0.9963 17.2
Application Note
Food & Beverage Testing
Author
Bruce D. Quimby
Agilent Technologies, Inc.
Abstract
Due to ongoing concerns with the price and availability of helium (He),
many laboratories are looking for alternative carrier gases for their gas
chromatography/mass spectrometry (GC/MS) methods. This application note
describes the conversion of a typical GC/MS method for the qualitative analysis
of flavor and fragrance compounds in essential oils from helium to hydrogen (H2
).
The Agilent 8890 GC coupled with the Agilent 5977C GC/MSD system were used
with hydrogen carrier gas and a new source that has been optimized for hydrogen
operation—the Agilent HydroInert source. Unlike most conventional electron
ionization (EI) sources, the HydroInert source provides excellent mass spectral
fidelity for flavor compounds when using hydrogen. To further increase confidence
in compound identification, deconvoluted mass spectra and linear retention indexes
(RI) from Agilent MassHunter Unknowns Analysis software were searched against
the NIST23 mass spectral library. Using the Agilent Method Translator tool, a column
and chromatographic conditions for hydrogen were chosen that allowed reduction
of the analysis time by a factor of 2.5 compared to the typical helium method. By
proper selection of instrument configuration and operating conditions, the system
with hydrogen carrier gas can generate results comparable to those with helium, but
with significantly reduced run time.
Qualitative Analysis of Essential Oils
Using GC/MS with Hydrogen Carrier
Gas and the Agilent HydroInert Source
Return to Table of Contents 101
2
Introduction
Essential oils are widely used as a source of flavors and
fragrances in both food and nonfood consumer products.
Quality control analysis of essential oils has long been
challenging due to the hundreds of terpenes and terpenoid
compounds that can be present in the oils. To address this,
methods using high-resolution capillary GC combined with
MS have typically been employed. Searching the acquired
mass spectra against libraries of flavor and fragrance
compounds can be performed for identification, but is usually
insufficient because many compounds, especially isomers,
give similar spectra. For this reason, RIs are often used as
a complement to spectral matching for more dependable
identifications. The measured RI of an unknown is used in
conjunction with the results of the spectral library search to
determine the best candidate for identification.
This application note describes the conversion of a typical
GC/MS method for the qualitative analysis of flavor and
fragrance compounds in essential oils from helium to
hydrogen. The two methods are then applied to the analysis
of two common essential oils—orange oil, of Brazilian origin,
and neroli oil—for comparison. The hydrogen method was
further evaluated using different EI source components to
determine the optimal source for maintaining spectral fidelity.
The conversion of a method from helium to hydrogen
carrier gas requires consideration of the chromatographic
parameters such as column choice, column flow, amount
injected, and temperature program rates.1
In addition, it is
important to consider the MS parameters of column flow rate
and MS source configuration. All these considerations and
parameters are included in the Agilent EI GC/MS Instrument
Helium to Hydrogen Carrier Gas Conversion User Guide.
1
When determining the chromatographic parameters for the
hydrogen method, it is desirable to have a method that has:
– Column dimensions that result in a high enough inlet
pressure for accurate flow control
– Similar chromatographic resolution to the original
helium method
– Maintenance of the same analyte elution order as the
original helium method
– Use of a column flow near the optimum for the column
diameter used
– Use of a column flow near the optimum for MS
source sensitivity
The Agilent Method Translator tool2-4 is a calculator
designed to greatly simplify this process. It was used in this
application note.
For the MS parameters, the column flow rate should be kept
within or near the optimum range of approximately 0.8 to
1.4 mL/min to maximize the MS response. Another important
consideration is the choice of MS EI source hardware.
The concern is that some analytes undergo reactions with
hydrogen in the source, changing their ion ratios and spectra,
thus reducing their library match scores (LMS), and possibly
resulting in misidentification. With the Agilent inert extractor
source used in the Agilent 5977 series GC/MSD systems,
this effect has been reduced in the past using an extractor
lens with a larger diameter, such as 9 mm. However, this only
partially addressed the problem, as many compounds such
as nitro compounds and some terpenes and terpenoids still
exhibited reactions. For this reason, Agilent developed the
HydroInert source5,6, which greatly reduces or eliminates
these reactions. To evaluate the effectiveness of the
HydroInert source, the qualitative analysis of the two oils was
carried out with the helium method using the standard 3 mm
inert extractor source and the hydrogen method using the
standard HydroInert source, which is equipped by default with
the 9 mm lens. In addition, the oils were analyzed with the
hydrogen method and a conventional inert extractor source
using both the 3 and 9 mm extractor lenses for comparison.
The next consideration is how to process the data files to
obtain the mass spectrum of each oil component and search
it against a library. In the past, this was done largely by
obtaining the apex or average spectrum over the peak then
subtracting a baseline spectrum taken next to the peak. The
resulting spectrum was then searched against the spectral
library. While this process is effective for handling a few peaks
that were relatively well resolved, it becomes overwhelming
with large numbers of peaks and/or overlapping peaks.
Fortunately, there is now a powerful solution for mass
spectral identification called Agilent MassHunter Unknowns
Analysis (MHUA), which is part of the Agilent MassHunter
Quantitative Analysis software suite. MHUA uses spectral
deconvolution to extract clean analyte spectra from the
complex overlapping peaks. The deconvolution and library
search processes are automated and take approximately 1 to
8 minutes per data file depending on the file size, library size,
computer hardware, and so on. The result is cleaner spectra
than with the previous approach, which therefore results in
higher LMS and greater confidence in peak identifications.7
102
3
A second major feature of MHUA is the ability to calculate the
RI for each peak. If the searched library has the appropriate
reference RI values associated with spectral entries, the
measured RI value for an unknown spectrum can be used to
filter the spectral search results. This is especially important
in the identification of essential oil components because of
spectral similarities. RI values were used in this application
note for this reason.
There are multiple mass spectral libraries available with RIs
for flavor and fragrance compounds. For example, the Adams
library8
has been used widely for many years for this analysis.
Recently, NIST released a newer version of their mass spectral
library (NIST23), which has numerous enhancements. Among
them are the incorporation of the entire Adams library and
the expansion of semistandard, nonpolar RI entries to cover
all EI spectra. "Semistandard, nonpolar" refers to phases such
as HP-5, DB-5, HP-5ms, and other 5% phenylmethyl silicone
phases.9
The new RI values are either experimental values, if
available, or artificial intelligence (AI)-generated values. Note
that the AI-generated values have better accuracy than the
previous "estimated" values. The new semistandard, nonpolar
values are of specific interest here because this type of
stationary phase is commonly used in essential oil analysis
with GC/MS. Also, these are the NIST23 values currently
usable with the RI function in MHUA. When the RI function of
MHUA is used, the experimental semistandard, nonpolar RI
values are used if available; if not, the AI-generated values are
used. Therefore, NIST23 is the library used here.
Experimental
Column selection
For the reference helium method, the column and conditions
chosen are like those frequently used in the past.8,10
A 30 m × 0.25 mm id, 0.25 μm Agilent J&W HP-5ms Ultra Inert
(UI) column (part number 19091S-433UI) was used with an
oven temperature program from 60 to 240 °C at 3 °C/min.
Although some of the older methods10 used constant
pressure control mode for column flow, constant flow mode
is far better in terms of MS performance. A constant column
flow rate of 1.0 mL/min of helium was used.
For the hydrogen method, a 20 m × 0.18 mm id, 0.18 μm
Agilent J&W HP-5ms UI column (part number 19091S-577UI)
was chosen. This column makes an excellent choice for
several reasons:
– The column dimensions provide an inlet pressure with
hydrogen that is high enough for accurate flow control.
– The column provides similar or better chromatographic
resolution compared to the helium method.
– The phase ratio is the same, helping maintain the same
analyte elution order as the original method.
– A column flow of hydrogen near the optimum for both
chromatographic separation and MS source sensitivity
can be used.
Method translation
The Method Translator tool is included as part of the Agilent
MassHunter acquisition software or can be downloaded
for standalone use from Agilent.com: https://www.agilent.
com/en/support/gas-chromatography/gccalculators.
The free download includes the Method Translator, Vapor
Volume Calculator, Pressure Flow Calculator, and Solvent
Vent Calculator tools, which are all useful when developing
GC methods.
After installation, the Method Translator is opened from
an icon on the desktop. The opened Method Translator is
shown in Figure 1. First, the chromatographic parameters
of the original helium method are entered in the left column
labeled Original Method Parameters. The carrier gas type
(He), column dimensions, column outlet pressure, and oven
temperature program ramp should be entered first. The
column outlet flow is then entered. As shown in Figure 1, the
other values such as phase ratio, inlet pressure, and so on, are
calculated automatically.
Next, the carrier gas type (H2
), column dimensions, and
column outlet pressure for the hydrogen method are entered
in the right side of the calculator under the Calculated Method
Parameters column.
After entry, the calculated hydrogen parameters are displayed.
In the upper-left corner, the calculated speed gain is shown
as 2.5877, meaning that the predicted retention times (RTs)
with the hydrogen method would be a factor of approximately
2.6 smaller than the helium method. The calculated oven
103
4
ramp rate for hydrogen would be 7.763 °C/min. Note that it
would be easier to have the oven ramp rate and the speed
gain closer to 7.5 and 2.5, respectively. This can be done
by selecting Speed gain and entering 2.5 into the field.
The parameters are recalculated, resulting in the desired
parameters. Figure 1 shows the results. Note that the
calculated flow for the hydrogen method shown in Figure 1
is 0.84004 mL/min. Before using the method, retention time
locking (RTL) was used to make the RT of n-pentadecane
in the hydrogen method precisely 2.5 times faster than that
with the helium method. This made comparison of RTs
easier. The resulting flow for the hydrogen method after
RTL was 0.958 mL/min. For library searching, this step is
not necessary as the RI calibration accounts for differences
in flow.
Figure 1. Agilent Method Translator tool, used to determine method parameters for conversion of the helium method to hydrogen.
104
5
MS source hardware
The standard inert extractor source with the 3 mm extractor
lens is an excellent choice for the helium method when
analyzing flavor and fragrance compounds, and was used in
this application note. For the hydrogen method, the HydroInert
source with the 9 mm extractor lens was used, as it reduces
in-source reactions with hydrogen and provides improved
peak shape. The hydrogen method was also run with a
conventional inert extractor source using both the 3 and 9
mm extractor lenses. These data were compared to that from
the HydroInert and helium results to identify components of
the oils that were most susceptible to in-source reactions by
comparing their spectra and LMS values. Figure 2 shows the
system configurations for the helium and hydrogen methods.
Chemicals and standards
In-house hydrogen with 99.9999% purity specification and
low individual specifications on water and oxygen was used
as the carrier gas for the hydrogen method. In-house helium
with similar specifications was used as the carrier gas for the
helium method.
Cold-pressed orange oil (Brazil origin) and neroli oil (Morocco
origin) were purchased from Sigma-Aldrich (Milwaukee, WI,
USA). The oils were diluted to 20:1 (v:v) in ethanol.
A custom RI calibration standard consisting of all the
n-alkanes from n-C5
to n-C40 plus n-C44 was purchased
from Ultra Scientific (now Agilent). All alkanes were at a
concentration of 500 ng/µL in n-hexane except n-C13, n-C18,
n-C22, n-C28, n-C31, and n-C39, which were at 1,000 ng/µL. The
standard was then diluted to 50:1 (v:v) in isooctane.
Figure 2. System configurations for the helium and hydrogen methods.
Liquid
injector
S/SL Inlet
(hydrogen) HydroInert source with 9 mm
extractor lens
EI source
20 m × 180 µm, 0.18 µm df
Agilent 8890
GC
Agilent 8890
GC
Liquid
injector
S/SL inlet
(helium) Inert extractor
with 3 mm
extractor lens
Agilent 5977C
GC/MSD
Agilent 5977C
GC/MSD
EI source
30 m × 250 µm, 0.25 µm df
Agilent HP-5ms UI Agilent HP-5ms UI
Helium configuration Hydrogen configuration
Table 1. GC and MS conditions for helium and hydrogen methods.
Method Parameters
Helium Method Hydrogen Method
Inlet EPC split/splitless
Mode Split 25:1
Column Flow 1.0 mL/min helium 0.958 mL/min hydrogen
Injection Volume 1.0 µL
Inlet Temperature 250 °C
Inlet Liner Agilent universal low pressure drop UI liner with wool
(p/n 5190-2295)
Column
Agilent J&W HP-5ms UI,
30 m × 0.25 mm, 0.25 µm
(p/n 19091S-433UI)
Agilent J&W HP-5ms UI,
20 m × 0.18 mm, 0.18 µm
(p/n 19091S-577UI)
Column Temperature
Program
60 °C (no hold)
3 °C/min to 240 °C
(no hold)
60 °C (no hold)
7.5 °C/min to 240 °C
(no hold)
Run Time 60 min 24 min
MSD Source Agilent inert extractor
(3 mm lens)
Agilent HydroInert source
(9 mm lens)
Transfer Line
Temperature
300 °C
Ion Source
Temperature
300 °C
Quadrupole
Temperature
150 °C
EM, Gain Mode 0.1
Mode Scan 40 to 400 m/z
TID, A/D Samples TID ON, 8 TID ON, 4
Solvent Delay 2.2 min 0.88 min
Tune etune.u
105
6
Results and discussion
Retention index calibration
The diluted RI calibration standard was run with both
methods. The chromatograms are shown in Figure 3.
Although the oil sample analysis is finished before n-C26,
the standard contains n-alkanes up to n-C40. Therefore, the
temperature ramps for both RI calibration methods were
extended to 300 °C and held until n-C40 eluted to prevent
carryover peaks in subsequent chromatograms. The red
arrow in each chromatogram indicates the normal end of
run for the oil methods. For determining the RT of the RI
calibration compounds, integrating the EIC for m/z 57 is
preferred over the total ion chromatogram (TIC), as it has a
better signal-to-noise ratio.
To use RI values in MHUA, a calibration file needs to be
created for each method. The file can be created as a .csv
file in Microsoft Excel, or as a text file in Microsoft Windows
Notepad. Figure 4 shows the calibration .rtc files created in
Notepad. The blue headers are not included in the files; they
are included here to indicate the entry format. Each entry
consists of the format name, CAS number, RI, and RT, and the
associated text file is then saved with a .rtc extension in the
filename. The text files are usually saved in either the library
directory or the directory containing the data files.
Figure 3. EICs at m/z 57 for the RI calibration standard. (A) Helium method; (B) hydrogen method.
0
0.2
0.4
0.6
0.8
1.0
1.2
1.4
1.6
1.8
2.0
2.2
2.4
2.6
2.8
3.0 7.757
15.485
20.532
26.737 1.985 3.036 4.446
6.068 9.432 11.049 12.596 14.075 16.828 18.116 29.390 19.348 21.666
22.754
23.806
24.817
25.792
27.648 28.532
2 3 4 5 6 7 8 9 10 11 12 13 14 15 16 17 18 19 20 21 22 23 24 25 26 27 28 29
0
0.1
0.2
0.3
0.4
0.5
0.6
0.7
0.8
0.9
1.0
1.1
1.2
1.3
1.4
1.5
1.6
1.7
1.8
1.9
19.377
4.804 38.694 7.485
11.051
15.137
23.577
51.260 27.622 31.489
35.177 66.686 73.266
42.049 45.257 48.320 54.087
56.792
59.400 61.918 64.339 68.953 71.138
4 6 8 10 12 14 16 18 20 22 24 26 28 30 32 34 36 38 40 42 44 46 48 50 52 54 56 58 60 62 64 66 68 70 72 74
13
28
22
18
30
9
14
10
11
12
20 19
17
16 15
29 23 21
25 26 27
24
31
Hydrogen
Agilent HP-5ms UI, 20 m × 0.18 mm id, 0.18 µm
240 °C (end of run for samples)
240 °C (end of run for samples)
Helium
Agilent HP-5ms UI, 30 m × 0.25 mm id, 0.25 µm
×105
A
B
Acquisition time (min)
Acquisition time (min)
Counts
×105
Counts
106
7
Brazilian orange oil
Figure 5 compares the TICs obtained with the helium and
hydrogen methods for the Brazilian orange oil sample.
Figure 5A shows the complete time range of elution, and
5B is an expanded view to better compare the peak shapes
and chromatographic resolution. As can be seen, by using
the Method Translator technique, the relative elution order
of peaks is maintained, as is the resolution. Many of the
larger peaks in the hydrogen chromatogram exhibited some
fronting. This was predicted by the Method Translator tool,
which indicated that the hydrogen setup has 36% of the
original column capacity of the helium method. However, the
chromatographic resolution is still about the same as the
helium method.
Figure 4. RI calibration text files (.rtc) used in Agilent MassHunter Unknowns Analysis.
Helium method
Name, CAS, RI, RT
Hydrogen method
Name, CAS, RI, RT
107
8
Figure 5. (A) Brazilian orange oil with helium and hydrogen methods showing full range of compound elution. (B) Expanded view of earlier elution time region.
0
0.1
0.2
0.3
0.4
0.5
0.6
0.7
0.8
0.9
1.0
1.1
1.2
1.3
1.4
4 6 8 10 12 14 16 18 20 22 24 26 28 30 32 34 36 38 40 42 44 46 48 50 52 54 56 58
0
0.2
0.4
0.6
0.8
1.0
1.2
1.4
1.6
1.8
2.0
2.2
2.4
1 2 3 4 5 6 7 8 9 10 11 12 13 14 15 16 17 18 19 20 21 22 23 24
Hydrogen
Agilent HP-5ms UI, 20 m × 0.18 mm id, 0.18 µm
Helium
Agilent HP-5ms UI, 30 m × 0.25 mm id, 0.25 µm
Brazilian orange oil
0
0.2
0.4
0.6
0.8
1.0
1.2
1.4
1.6
1.8
2.0
2.2
1 2 3 4 5 6 7 8 9 10 11 12
0
0.2
0.4
0.6
0.8
1.0
1.2
1.4
3 4 5 6 7 8 9 10 11 12 13 14 15 16 17 18 19 20 21 22 23 24 25 26 27 28 29 30
Brazilian orange oil, helium method
Brazilian orange oil, hydrogen method
×106
×106
×106
×106
A
B
Acquisition time (min)
Counts Counts Counts Counts
108
9
Neroli oil
Figure 6 shows the TICs for the neroli oil run with the helium
and hydrogen methods. As with the orange oil, the relative
elution order of peaks is maintained, as is the resolution. The
reduced column capacity of the hydrogen method is again
evident in the increased fronting of the large peak at 4.6
minutes in the hydrogen chromatogram.
Peak identification with MassHunter Unknowns Analysis
The parameters used with MHUA are listed in Table 2. If the
library has appropriate RI values for the spectrum entries, RIs
can be used as a filter for library hits. The program uses RI if
an RI calibration filename is entered in the RT calibration file
box. A more detailed description for setting up and running an
analysis is shown in the Appendix. Also, an excellent source
of information about MHUA is available in a video on the
Agilent YouTube channel.7
With the settings listed in Table 2 and a data file analyzed, the
program will deconvolute the entire scan file and determine
where each detectable peak (component) is. It will then take
the deconvoluted (cleaned) spectrum of each component and
search it against the library (NIST23). The library entry that
best matches the spectrum of the component is checked to
see if it exceeds the minimum match factor parameter of 70.
If it does, it is next checked to see if the measured RI of the
component falls within ± the penalty free range, in this case
± 10 seconds. With the RT mismatch penalty set to Additive
and the maximum RT penalty set to 100, if the difference
between the measured RT and the library RI (converted to RT)
is greater than 10 seconds, the entry is completely discarded.
If the difference is less than 10 seconds and the LMS is
greater than 70 and higher than the other possible hits, the hit
is included in the results table.
Figure 6. Neroli oil analyzed with (A) helium and (B) hydrogen methods.
0
0.2
0.4
0.6
0.8
1.0
1.2
1.4
1.6
1.8
2.0
2.2
2.4
2.6
2.8
3.0
3.2
3.4
4 6 8 10 12 14 16 18 20 22 24 26 28 30 32 34 36 38 40 42 44 46 48 50 52 54 56 58
Helium
Agilent HP-5ms UI, 30 m × 0.25 mm id, 0.25 µm
0
0.5
1.0
1.5
2.0
2.5
3.0
3.5
4.0
4.5
5.0
5.5
6.0
6.5
7.0
2 3 4 5 6 7 8 9 10 11 12 13 14 15 16 17 18 19 20 21 22 23
Hydrogen
Agilent HP-5ms UI, 20 m × 0.18 mm id, 0.18 µm
Neroli oil
×106
A
Acquisition time (min)
Acquisition time (min)
Counts
×106
B
Counts
109
10
Table 2. Agilent MassHunter Unknowns Analysis method parameters.
Parameter Setting
RT Window Size Factor 10, 25, 50, 100, 200, 400, 600, 800
Library NIST23.L
RT Penalty function Trapezoidal
RT Range 10 s
Penalty Free Range 10 s
RT Mismatch Penalty Additive
Maximum RT Penalty 100
Minimum Match Factor 70
Once the entire data file has been processed, the results
can be reviewed. Figure 7 shows the results for the Brazilian
orange oil with the helium method. Left-clicking on the
compound name for one of the listed results displays the
deconvoluted component spectrum head to tail with the
library spectrum.
Figure 7. Agilent MassHunter Unknowns Analysis results for Brazilian orange oil run with the helium method.
Identification results
TIC chromatogram
Component spectrum
NIST 23 library spectrum
The selected component peak at 16.989 minutes is
highlighted in red in the TIC chromatogram, which can be
zoomed in for closer inspection. The five most abundant
ions are extracted and overlaid in the box to the left of
the spectrum display. This is to allow inspection of the
peak shapes and apex retention times. If the apex RT or
shape of one of the extracted ions is substantially different
from the others, this suggests that there might be an
interference, which should be considered when interpreting
the identification.
In practice, reviewing the results consists of going down the
list of hits and looking at the Match Factor (Library Match
Score), Delta RI, and Base Peak Area. Using the peak at
16.989 as an example, the spectrum has a high-quality match
for D-carvone of 98.2 listed, and the head-tail component and
library spectra visually match well. The overlay of EICs of the
principal ions all have the same shape and apex RT. The delta
RI value of 2, which is the difference between the measured
RI for the peak and that from the library, is small at 2. Finally,
the base peak area and observed peak size in the TIC
chromatogram indicate that the response is large enough to
produce good-quality spectra. From these observations, the
identification of D-carvone is confirmed with high confidence.
110
11
In contrast, the peak at 17.6172, identified as
p-mentha-1(7),8(10)-dien-9-ol, has a low LMS of 74.6, a
larger delta RI of 4, one of the EICs has a noticeably different
apex RT, and the base peak area is small—approximately
190 times smaller than the D-carvone peak. This would be
a low-confidence identification. If the reviewer decides it
should not be reported, the hit can be removed from the
results by right-clicking the name and selecting Delete
Components/Hits.
If an identification is questioned based on other information,
the reviewer can right-click the name in the results table and
select Show Alternate Hits. This will display a list of the other
spectra in the library that also met the LMS and RI criteria,
but with an LMS less than the listed Best Hit. This is useful
as sometimes the LMS of the lesser hits is only a fraction of
a point smaller. If desired, the reviewer can select one of the
alternate hits and set it as the identification.
This review process is used to evaluate all the hits. Once
completed, the reviewed analysis can be saved and a report
can be generated if desired.
Evaluating in-source reactions with hydrogen
While the inert extractor source with the 3 mm lens is
standard for use with helium, it is not the source of choice
for use with hydrogen carrier. The metal surfaces inside the
source tend to catalyze reactions between hydrogen and
some analyte molecules in the source, resulting in peak
tailing and spectral changes for some compounds. In the
past, substituting the 9 mm extractor lens for the 3 mm lens
was used as it reduced the tailing and spectral changes to
some degree, but did not eliminate them. For this reason, the
HydroInert source was developed.
In this section, the spectra of carvone oxide (CAS number
33204-74-9) obtained with hydrogen using the HydroInert
source, the inert extractor source with the 3 and 9 mm lenses,
and with helium are compared to illustrate the effects of
source reactivity. Several other examples are presented in
the Appendix.
Figure 8 shows the chromatograms and spectra of
the carvone oxide peak with the helium and hydrogen
methods under the optimized conditions. The library
reference spectrum from NIST23 is shown upside down for
comparison. With both methods, the deconvoluted spectra
have high LMS values of > 95, demonstrating the excellent
spectral fidelity provided by the HydroInert source with
hydrogen carrier gas.
Figure 8. (A) Chromatogram and spectrum of the carvone oxide peak with the helium method. (B) Chromatogram and spectrum of the carvone oxide peak with
the hydrogen method and Agilent HydroInert source.
3 mm Inert extractor, He
Component RT: 18.4298
40 50 60 70 80 90 100 110 120 130 140 150 160 170
Counts Counts
-1
-0.8
-0.6
-0.4
-0.2
0
0.2
0.4
0.6
0.8
43.0
43.1 123.1 85.1 67.1
95.1
67.0 85.0
109.1
123.0 95.0
55.1
55.0 109.0
137.1 148.0
137.0 148.0 166.0
165.0
3 mm Inert extractor, He
LMS = 95.5
9 mm HydroInert H2
LMS = 95.8
Carvone oxide Carvone oxide
16.5 17.0 17.5 18.0 18.5 19.0 19.5 20.0 20.5 21.0
0
0.2
0.4
0.6
0.8
1.0
1.2
1.4
1.6
1.8
2.0
2.2
2.4
2.6
16.2812
16.4233
16.9890
16.6057
16.7963
17.4855
17.6172
17.8714
18.1399
18.2331
18.4298
18.7572
18.9074
19.2663
19.5336
20.2660
21.2728 21.0191
9 mm HydroInert, H2
6.6 6.8 7.0 7.2 7.4 7.6 7.8 8.0 8.2 8.4
Counts
0
0.5
1.0
1.5
2.0
2.5
3.0
3.5
4.0
6.6160
6.8418
8.4586
7.2896
7.5229
7.7307
7.5880
7.3999
7.3203
6.5497
7.0261
8.5487
7.8424
7.1778
8.1341
6.6932
6.7527
Component RT: 7.3999
40 50 60 70 80 90 100 110 120 130 140 150 160 170
Counts
-1.2
-1.0
-0.8
-0.6
-0.4
-0.2
0.2
0
0.4
0.6
0.8
1.0
43.0
43.0 67.1
84.9 123.1
67.0 85.0
95.1
95.0 123.0
55.1 109.1
55.0 109.0
137.1
137.0
147.9
148.0
165.9
166.0
×105
×102
×105
×102
A B
Acquisition time (min) Acquisition time (min)
Mass-to-charge (m/z) Mass-to-charge (m/z)
111
12
For comparison, Figure 9 shows the spectra of the carvone
oxide peak with hydrogen carrier using the HydroInert source
and the inert extractor source with both the 9 and 3 mm
extractor lenses.
With the 9 mm inert extractor lens shown in the spectrum in
Figure 9B, the LMS value is still a respectable 91.2. However,
there is clear evidence of some spectral changes. Most
notably, the ions 82 and 108 have increased in abundance
relative to the rest of the ions in the spectrum. While the
degree of spectral fidelity is still useful, it demonstrates that
in-source reactions, albeit limited, are occurring.
In contrast, the spectrum with the 3 mm inert extractor
lens and hydrogen carrier is significantly changed. The
spectrum is changed to the extent that the LMS for matching
carvone oxide is below the 70 cutoff and thus is not listed,
even in the alternate hits list. The search identified the peak
as (2,6,6-trimethylbicyclo[3.1.1]heptan-3-yl)methanamine
(CAS 61299-72-7), also known as pinane-3-(methylamine).
Note that with an LMS value of 84.8 and a close RI match
with a delta RI of only –1, this identification looks plausible
but is incorrect.
Component RT: 7.4187
43.0
82.0 67.0 43.0
123.0 95.0
67.0 85.0
108.0 55.0
95.0 123.0 55.0 109.0
137.0
137.0
151.0 166.0
166.0
9 mm Inert extractor, H2
LMS = 91.2
Component RT: 7.4171
40 50 60 70 80 90 100 110 120 130 140 150 160 170
82.0
95.0
82.0
67.0 108.0 93.0
67.0
43.0
41.0
123.0
107.0
54.0
55.0
135.0
135.0
150.0
121.0 152.0
3 mm Inert extractor, H2
LMS = 84.8
(but wrong compound)
Component RT: 7.3999
40 50 60 70 80 90 100 110 120 130 140 150 160 170
-1.2
-1.0
-0.8
-0.6
-0.4
-0.2
0
0.2
0.4
0.6
0.8
1.0
-1.2
-1.0
-0.8
-0.6
-0.4
-0.2
0
0.2
0.4
0.6
0.8
1.0
43.0
43.0 67.1
84.9 123.1
67.0 85.0
95.1
95.0 123.0
55.1 109.1
55.0 109.0
137.1
137.0
147.9
148.0
165.9
166.0
9 mm HydroInert, H2
LMS = 95.8
×102
×102
A
B
CCounts Counts
-1.2
-1.0
-0.8
-0.6
-0.4
-0.2
0
0.2
0.4
0.6
0.8
1.0
×102
Counts
Mass-to-charge (m/z)
40 50 60 70 80 90 100 110 120 130 140 150 160 170
Mass-to-charge (m/z)
Mass-to-charge (m/z)
Figure 9. (A) Spectrum of the carvone oxide with hydrogen carrier and the
Agilent HydroInert source. The reference spectrum is carvone oxide from
NIST23. (B) Spectrum with an Agilent inert extractor source and a 9 mm
lens. The reference spectrum is carvone oxide from NIST23. (C) Spectrum
with an Agilent inert extractor source and a 3 mm lens. The reference
spectrum is (2,6,6-trimethylbicyclo[3.1.1]heptan-3-yl)methanamine
from NIST23.
112
13
To further investigate the nature of the in-source reaction with
the 3 mm inert extractor source, the data file was reanalyzed
with MHUA using the same parameters, except not using the
RI match criteria. This would list the best hits solely on LMS.
If the carvone oxide is reacting with hydrogen in the source
to produce a reaction product, the spectral search may reveal
what it is. Figure 10 shows the spectrum obtained with (A) the
3 mm lens at the carvone oxide RT compared with (B) that of
the best match and (C) the carvone oxide library spectrum.
Note that the spectrum obtained with the 3 mm inert
extractor source looks very much like a combination of that
of carvone oxide and 3-hydroxy-2-methyl-5-(prop-1-en-2-yl)
cyclohexanone. Examining the structures in Figure 10, it
appears that the epoxide structure of carvone oxide reacts
with hydrogen to form the OH group.
This example clearly illustrates the perils of using a GC/MS
source that allows reactions between hydrogen and analytes,
and why the HydroInert source is the best choice when using
hydrogen carrier gas. Several other examples are shown in
the Appendix.
Figure 10. (A) Spectrum obtained with the 3 mm lens at the carvone oxide RT. (B) NIST23 library reference spectrum for the best match when searched without RI
filtering, 3-hydroxy-2-methyl-5-(prop-1-en-2-yl)cyclohexanone. (C) NIST23 library reference spectrum for carvone oxide.
30
30
40 50 60 70 80 90 100 110 120 130 140 150 160 170 180 190 200 210 220 230
0
0.1
0.2
0.3
0.4
0.5
0.6
0.7
0.8
0.9
1 43.0
67.0
85.0
123.0 95.0
55.0 81.0
109.0
71.0
105.0 137.0 148.0
40 50 60 70 80 90 100 110 120 130 140 150 160 170 180 190 200 210 220 230
0
0.1
0.2
0.3
0.4
0.5
0.6
0.7
0.8
0.9
1 43.0
108.0 82.0
67.0
54.0
79.0 95.0
71.0
58.0 122.0 135.0 150.0
NIST23 spectrum of best library match
when searched without RI
NIST23 spectrum of carvone oxide
A
B
C Counts Counts Counts
×103
×105
×103
HO
CH3
CH2
H3
C
O
O CH3
CH2
H3
C
O
Carvone oxide
3-Hydroxy-2-methyl-5-(prop-1-en-2-yl)cyclohexanone
30 40 50 60 70 80 90 100 110 120 130 140 150 160 170 180 190 200 210 220 230
0
0.1
0.2
0.3
0.4
0.5
0.6
0.7
0.8
0.9
82.0
108.0 67.0 93.0
43.0
123.0 54.0
135.0
150.0
166.0 192.0 222.0
Spectrum at carvone oxide RT
113
14
Analysis results for Brazilian orange and neroli oils
Table 3 presents the results of the analysis of the Brazilian
orange oil with both the helium method using the 3 mm inert
extractor source and the hydrogen method using the 9 mm
HydroInert source. Table 4 presents the results for neroli oil
with the same methods.
The results were reviewed to address questions relevant to
converting the method helium to hydrogen carrier gas:
– RIs compared to NIST23: The RIs measured with both
methods closely matched those in the NIST23 library for
most compounds. However, it should be recognized that
the RI recognition window used in MassHunter Unknowns
Analysis limits the maximum delta RI when listing hits. The
second consideration is that if the NIST23 RI value is an
AI-predicted value instead of a true experimental value, the
errors, and thus the delta RIs, can be larger.
– Comparing RIs between helium and hydrogen methods:
As seen in the columns listing the difference between the
RI measured with helium and that with hydrogen (labeled
RI He-RI H2
), the agreement of the RIs measured with
both methods is good. The only exceptions are the earliest
peaks and those such as linalool and D-limonene that are
chromatographically overloaded, thus shifting their RT.
This is one of the benefits of using the Method Translator
tool to choose the chromatographic parameters for the
hydrogen method, because it maintains the same relative
elution order for analytes and RI calibrators between the
two methods.
– LMS versus NIST23: The deconvolution process in general
yields cleaner spectra, which results in improved LMS
scores when compared to previous approaches. Looking
at the column of LMS scores for the helium results,
most of them are > 85. The smaller values can result
from smaller responding compounds, overlapping peaks
causing spectral interferences, or search results where the
analyte is not in the library and an incorrect hit is listed.
– Comparing LMS between helium and hydrogen methods:
The columns listing the difference between the LMS
measured with helium and that with hydrogen (labeled
LMS He-LMS H2
) shows that, in general, there is good
agreement between the two methods. The exceptions
where the hydrogen method values are significantly
lower are either due to lower signal response or spectral
interference from overlapping peaks. In general, the
signal-to-noise ratios obtained with hydrogen are two to
five times less than with helium, and this is reflected in
lower LMS scores for the smallest peaks.
114
15
Table 3. Analysis results for the Brazilian orange oil with both the helium and hydrogen methods. (LR = low response; Int = interference.)
Compound Name CAS Lib RI
Helium Hydrogen with Hydroinert RI HeRI H2
LMS HeLMS H2 RT RI Delta RI LMS RT RI Delta RI LMS
Ethane, 1,1-diethoxy- 105-57-7 726 2.445 723 3 98 1.061 732 -6 96 -9 2
Nonane 111-84-2 900 4.807 900 0 95 1.983 900 0 91 0 4
(1R)-2,6,6-Trimethylbicyclo[3.1.1]hept-2-ene 7785-70-8 932 5.688 933 -1 99 2.332 933 -1 97 0 2
1-Heptanol 111-70-6 970 6.566 966 4 98 2.699 968 2 95 -2 2
Bicyclo[3.1.0]hexane, 4-methylene-1-(1-methylethyl)- 3387-41-5 974 6.757 973 1 98 2.759 974 0 98 -1 0
beta-Myrcene 123-35-3 991 7.240 991 0 97 2.953 992 -1 95 -1 2
Octanal 124-13-0 1,003 7.616 1,004 -1 98 3.116 1,006 -3 97 -2 1
3-Carene 13466-78-9 1,011 7.883 1,011 0 98 3.211 1,012 -1 89 -1 9
D-Limonene 5989-27-5 1,031 8.682 1,034 -3 99 3.562 1,037 -6 99 -3 0
1-Methylbicyclo[2.2.1]heptan-exo-2-ol 766-25-6 1,039 8.796 1,037 2 76 3.593 1,039 0 74 -2 2
trans-Sabinene hydrate 17699-16-0 1,070 9.837 1,066 4 74 3.985 1,067 3 77 -1 -3
1-Octanol 111-87-5 1,070 9.919 1,068 2 98 4.016 1,070 0 97 -2 1
cis-Linalool oxide 5989-33-3 1,074 10.039 1,072 2 94 4.063 1,073 1 94 -1 0
trans-Linalool oxide (furanoid) 34995-77-2 1,086 10.630 1,088 -2 95 4.297 1,089 -3 94 -1 0
Benzene, 1-methyl-4-(1-methylethenyl)- 1195-32-0 1,090 10.665 1,089 1 91 4.306 1,090 0 92 -1 -1
Epoxymyrcene,6,7- 29414-55-9 1,090 10.804 1,093 -3 73 4.365 1,094 -4 79 -1 -6
Linalool 78-70-6 1,099 11.051 1,100 -1 97 4.462 1,101 -2 95 -1 1
Nonanal 124-19-6 1,104 11.232 1,104 0 96 4.533 1,105 -1 86 -1 10 LR
cis-Pinen-3-ol 1010292-85-2 1,108 11.324 1,107 1 81 4.574 1,108 0 73 -1 8
2-Cyclohexen-1-ol, 1-methyl-4-(1-methylethenyl)-,
trans- 7212-40-0 1,123 11.872 1,120 3 95 4.799 1,122 1 96 -2 -1
5-Undecene, 4-methyl- 143185-91-5 1,132 12.217 1,129 3 74 4.925 1,130 2 71 -1 4
7-Oxabicyclo[4.1.0]heptane,
1-methyl-4-(1-methylethenyl)- 1195-92-2 1,133 12.401 1,133 0 86 5.003 1,134 -1 90 -1 -4
cis-p-Mentha-2,8-dien-1-ol 3886-78-0 1,133 12.462 1,135 -2 79 5.042 1,137 -4 89 -2 -10
(+)-(E)-Limonene oxide 6909-30-4 1,139 12.586 1,138 1 97 5.082 1,139 0 98 -1 -1
Cyclohexanol, 1-methyl-4-(1-methylethenyl)-, cis- 7299-41-4 1,144 12.826 1,143 1 95 5.171 1,145 -1 93 -2 1
Cyclohexanol, 1-methyl-4-(1-methylethenyl)-, trans- 7299-40-3 1,161 13.610 1,163 -2 88 5.480 1,164 -3 83 -1 4
Bicyclo[3.3.0]octan-2-one, 7-methylene- 1000151-92-1 1,166 13.822 1,168 -2 84 5.578 1,170 -4 64 -2 19 Int
1-Nonanol 143-08-8 1,173 13.921 1,170 3 98 5.603 1,171 2 96 -1 2
Ethanone, 1-(4-methylphenyl)- 122-00-9 1,183 14.452 1,183 0 93 5.822 1,185 -2 95 -2 -2
p-Mentha-1(7),8-dien-2-ol 35907-10-9 1,186 14.596 1,187 -1 97 5.873 1,188 -2 96 -1 1
alpha-Terpineol 98-55-5 1,189 14.730 1,190 -1 95 5.928 1,191 -2 94 -1 1
Bicyclo[3.1.1]hept-2-ene-2-methanol, 6,6-dimethyl- 515-00-4 1,195 14.966 1,196 -1 84 6.018 1,197 -2 86 -1 -2
Decanal 112-31-2 1,206 15.374 1,206 0 94 6.173 1,206 0 93 0 1
Acetic acid, octyl ester 112-14-1 1,210 15.663 1,212 -2 91 6.287 1,213 -3 79 -1 12 LR, Int
Dihydro carveol, iso- 18675-35-9 1,212 15.756 1,215 -3 85 6.334 1,216 -4 78 -1 7
trans-Carveol 1197-07-5 1,217 15.946 1,219 -2 95 6.427 1,221 -4 96 -2 -1
trans-3(10)-Caren-2-ol 1010151-66-5 1,227 16.281 1,227 0 89 6.550 1,229 -2 85 -2 4
2-Cyclohexen-1-ol, 2-methyl-5-(1-methylethenyl)-, cis- 1197-06-4 1,229 16.423 1,230 -1 96 6.616 1,232 -3 97 -2 -1
2,4-Cycloheptadien-1-one, 2,6,6-trimethyl- 503-93-5 1,238 16.606 1,235 3 81 6.693 1,237 1 73 -2 8
Benzaldehyde, 4-(1-methylethyl)- 122-03-2 1,239 16.796 1,239 0 73 6.753 1,241 -2 58 -2 14 LR
D-Carvone 2244-16-8 1,246 16.989 1,244 2 98 6.842 1,246 0 98 -2 0
Geraniol 106-24-1 1,255 17.486 1,255 0 72 7.026 1,257 -2 77 -2 -5
p-Mentha-1(7),8(10)-dien-9-ol 29548-13-8 1,262 17.617 1,258 4 75 7.070 1,259 3 73 -1 2
115
16
Compound Name CAS Lib RI
Helium Hydrogen with Hydroinert RI HeRI H2
LMS HeLMS H2 RT RI Delta RI LMS RT RI Delta RI LMS
4-Cyclohexylidenebutyraldehyde 937-59-7 1,268 17.871 1,264 4 75 7.178 1,266 2 76 -2 -2
2-Cyclohexen-1-one, 3-methyl-6-(1-methylethenyl)-,
(S)- 16750-82-6 1,270 18.140 1,271 -1 91 7.290 1,272 -2 83 -1 7
1-Cyclohexene-1-carboxaldehyde,
4-(1-methylethenyl)- 2111-75-3 1,273 18.233 1,273 0 97 7.320 1,274 -1 84 -1 14 LR
Carvone oxide 33204-74-9 1,279 18.430 1,278 1 96 7.400 1,279 0 96 -1 0
Pinocarvyl acetate, cis- 73366-18-4 1,285 18.757 1,285 0 80 7.523 1,286 -1 82 -1 -2
Verbenyl acetate, trans- 1203-21-0 1,291 18.907 1,289 2 79 7.588 1,290 1 80 -1 0
p-Mentha-1,8-dien-7-ol 536-59-4 1,297 19.266 1,297 0 95 7.731 1,298 -1 93 -1 2
2-Propanol, 1-[(1-ethynylcyclohexyl)oxy]- 54644-17-6 1,303 19.534 1,304 -1 77 7.842 1,305 -2 79 -1 -1
1,2-Cyclohexanediol, 1-methyl-4-(1-methylethenyl)- 1946-00-5 1,321 20.266 1,321 0 80 8.134 1,323 -2 84 -2 -4
(1S,4R,5R)-1,3,3-Trimethyl-2-oxabicyclo[2.2.2]octan5-yl acetate 81781-24-0 1,343 21.019 1,339 4 83 8.459 1,342 1 82 -3 1
exo-2-Hydroxycineole acetate 57709-95-2 1,344 21.273 1,345 -1 86 8.549 1,347 -3 89 -2 -2
1-Cyclohexene-1-methanol, 4-(1-methylethenyl)-,
formate 29621-55-4 1,356 21.612 1,353 3 87 8.664 1,354 2 90 -1 -4
2-Cyclohexen-1-ol, 2-methyl-5-(1-methylethenyl)-,
acetate, (1R-cis)- 7111-29-7 1,358 21.856 1,359 -1 80 8.775 1,361 -3 79 -2 1
Copaene 3856-25-5 1,376 22.546 1,375 1 91 9.024 1,376 0 84 -1 6
2-Methyl-4-(2,6,6-trimethylcyclohex-2-enyl)but-3-en2-ol 56763-65-6 1,406 23.737 1,404 2 83 9.528 1,406 0 85 -2 -2
cis-β-Copaene 18252-44-3 1,432 24.728 1,428 4 93 9.897 1,429 3 89 -1 3
Sesquicineole, 7-epi-1,2-dehydro- 149067-90-3 1,471 26.498 1,472 -1 78 10.618 1,473 -2 79 -1 -1
3-Tetradecen-5-yne, (E)- 74744-44-8 1,488 27.032 1,485 3 72 10.842 1,487 1 73 -2 -1
Valencene 4630-07-3 1,492 27.311 1,492 0 97 10.930 1,493 -1 96 -1 0
Caryophyllene oxide 1139-30-6 1,581 30.784 1,582 -1 73 12.320 1,582 -1 81 0 -7
1,5,9-Cyclododecanetriol 2938-55-8 2,007 45.413 2,005 2 72 18.190 2,006 1 76 -1 -4
3-Eicosyne 61886-66-6 2,032 46.366 2,036 -4 75 18.573 2,037 -5 76 -1 -2
Uvidin C, diacetate 1000501-90-0 2,107 48.516 2,107 0 75 19.432 2,107 0 73 0 2
(9E,11E)-Octadecadienoic acid 544-71-8 2,237 52.406 2,241 -4 74 20.997 2,241 -4 73 0 2
Incensole oxide, acetate 1000513-23-1 2,270 53.383 2,275 -5 72 21.395 2,276 -6 69 -1 3
116
17
Compound Name CAS Lib RI
Helium Hydrogen with Hydroinert RI HeRI H2
LMS HeLMS H2 RT RI Delta RI LMS RT RI Delta RI LMS
Ethane, 1,1-diethoxy- 105-57-7 726 2.445 723 3 98 1.060 732 -6 97 -9 1
3-Hexen-1-ol 544-12-7 856 3.982 851 5 93 1.685 856 0 80 -5 13 LR
1-Hexanol 111-27-3 868 4.184 863 5 95 1.762 867 1 92 -4 3
Bicyclo[3.1.0]hex-2-ene, 2-methyl-5-(1-
methylethyl)- 2867-05-2 929 5.501 926 3 98 2.258 926 3 92 0 6
(1R)-2,6,6-Trimethylbicyclo[3.1.1]hept-2-ene 7785-70-8 932 5.688 933 -1 98 2.334 933 -1 98 0 1
Camphene 79-92-5 952 6.087 948 4 98 2.496 949 3 97 -1 1
Benzaldehyde 100-52-7 962 6.380 959 3 98 2.628 961 1 96 -2 2
Bicyclo[3.1.1]heptane, 6,6-dimethyl-2-
methylene-, (1S)- 18172-67-3 978 6.899 978 0 97 2.824 980 -2 98 -2 0
m-Mentha-4,8-diene, (1S,3S)-(+)- 5208-51-5 983 7.047 984 -1 82 2.875 985 -2 77 -1 5
beta-Myrcene 123-35-3 991 7.240 991 0 97 2.952 992 -1 97 -1 0
Cyclohexane, 1-methylene-4-(1-methylethenyl)- 499-97-8 1,004 7.667 1,005 -1 98 3.121 1,006 -2 97 -1 1
cis-Anhydrolinalool oxide 54750-69-5 1,007 7.775 1,008 -1 93 3.166 1,009 -2 79 -1 14 LR, Int
3-Carene 13466-78-9 1,011 7.874 1,011 0 97 3.203 1,012 -1 97 -1 1
1,3-Cyclohexadiene, 1-methyl-4-(1-methylethyl)- 99-86-5 1,017 8.082 1,017 0 97 3.285 1,018 -1 97 -1 0
D-Limonene 5989-27-5 1,031 8.536 1,029 2 99 3.476 1,031 0 99 -2 0
1,3,6-Octatriene, 3,7-dimethyl-, (Z)- 3338-55-4 1,038 8.782 1,036 2 97 3.562 1,037 1 97 -1 0
trans-beta-Ocimene 3779-61-1 1,049 9.174 1,047 2 97 3.724 1,049 0 97 -2 0
gamma-Terpinene 99-85-4 1,060 9.540 1,058 2 99 3.860 1,058 2 99 0 0
trans-Sabinene hydrate 17699-16-0 1,070 9.843 1,066 4 90 3.994 1,068 2 81 -2 9
cis-Linalool oxide 5989-33-3 1,074 10.047 1,072 2 98 4.071 1,073 1 96 -1 2
1,4-Undecadiene, (Z)- 55976-14-2 1,080 10.241 1,077 3 81 4.138 1,078 2 80 -1 1
Cyclohexene, 1-methyl-4-(1-methylethylidene)- 586-62-9 1,088 10.638 1,088 0 98 4.294 1,089 -1 98 -1 0
Linalool 78-70-6 1,099 11.285 1,106 -7 98 4.618 1,111 -12 98 -5 0
Phenylethyl alcohol 60-12-8 1,116 11.611 1,114 2 98 4.725 1,117 -1 96 -3 1
2-Cyclohexen-1-ol, 1-methyl-4-(1-methylethyl)-,
cis29803-82-5 1,122 11.938 1,122 0 93 4.837 1,124 -2 93 -2 0
2,4,6-Octatriene, 2,6-dimethyl-, (E,Z)- 7216-56-0 1,131 12.231 1,129 2 99 4.935 1,130 1 99 -1 0
2-Isopropylimidazole 36947-68-9 1,132 12.318 1,131 1 71 4.973 1,132 0 70 -1 1
cis-p-Mentha-2,8-dien-1-ol 3886-78-0 1,133 12.460 1,134 -1 83 5.029 1,136 -3 82 -2 1
Benzyl nitrile 140-29-4 1,144 12.594 1,138 6 98 5.095 1,140 4 94 -2 5
Myroxide 28977-57-3 1,140 12.747 1,142 -2 92 5.144 1,143 -3 71 -1 20 LR, Int
Terpinen-4-ol 562-74-3 1,177 14.182 1,177 0 96 5.709 1,178 -1 96 -1 1
Benzenemethanol, alpha,alpha,4-trimethyl- 1197-01-9 1,183 14.515 1,185 -2 91 5.864 1,187 -4 90 -2 1
alpha-Terpineol 98-55-5 1,189 14.790 1,192 -3 99 5.972 1,194 -5 99 -2 0
1,3-Cyclohexadiene-1-carboxaldehyde,
2,6,6-trimethyl- 116-26-7 1,201 15.153 1,200 1 78 6.099 1,202 -1 79 -2 -2
(3E,5E)-2,6-Dimethylocta-3,5,7-trien-2-ol 206115-88-0 1,202 15.481 1,208 -6 88 6.229 1,210 -8 89 -2 -1
Acetic acid, octyl ester 112-14-1 1,210 15.648 1,212 -2 92 6.285 1,213 -3 89 -1 3
Benzofuran, 2-ethenyl- 7522-79-4 1,220 15.994 1,220 0 89 6.428 1,221 -1 86 -1 4
2,6-Octadien-1-ol, 3,7-dimethyl-, (Z)- 106-25-2 1,228 16.337 1,228 0 97 6.577 1,230 -2 98 -2 0
Neral 106-26-3 1,240 16.860 1,241 -1 90 6.776 1,242 -2 88 -1 2
Carvone 99-49-0 1,242 16.966 1,243 -1 87 6.821 1,245 -3 86 -2 2
Linalyl acetate 115-95-7 1,257 17.587 1,258 -1 95 7.079 1,260 -3 92 -2 3
Citral 5392-40-5 1,273 18.124 1,270 3 93 7.283 1,272 1 89 -2 4
Table 4. Analysis results for neroli oil with both the helium and hydrogen methods. (LR = low response; Int = interference.)
117
18
Compound Name CAS Lib RI
Helium Hydrogen with Hydroinert RI HeRI H2
LMS HeLMS H2 RT RI Delta RI LMS RT RI Delta RI LMS
2,6-Octadien-1-ol, 3,7-dimethyl-, formate, (Z)- 2142-94-1 1,282 18.575 1,281 1 89 7.452 1,282 0 89 -1 0
Levo-bornyl acetate 5655-61-8 1,285 18.780 1,286 -1 99 7.534 1,287 -2 95 -1 4
Indole 120-72-9 1,294 18.998 1,291 3 99 7.637 1,293 1 99 -2 0
Benzene, (2-nitroethyl)- 6125-24-2 1,302 19.280 1,298 4 89 7.746 1,299 3 86 -1 3
Geranyl formate 105-86-2 1,300 19.483 1,303 -3 91 7.811 1,303 -3 91 0 1
δ-EIemene 20307-84-0 1,338 20.958 1,338 0 86 8.392 1,338 0 96 0 -10
Methyl anthranilate 134-20-3 1,343 21.020 1,339 4 94 8.439 1,341 2 98 -2 -4
alpha-Terpinyl acetate 80-26-2 1,350 21.445 1,349 1 97 8.593 1,350 0 96 -1 2
6-Octen-1-ol, 3,7-dimethyl-, acetate 150-84-5 1,354 21.647 1,354 0 84 8.669 1,354 0 81 0 2
2,6-Octadien-1-ol, 3,7-dimethyl-, acetate, (Z)- 141-12-8 1,364 22.133 1,366 -2 99 8.881 1,367 -3 99 -1 0
Copaene 3856-25-5 1,376 22.543 1,375 1 82 9.026 1,376 0 80 -1 2
Geranyl acetate 105-87-3 1,382 22.966 1,385 -3 98 9.222 1,387 -5 98 -2 0
levo-β-Elemene 515-13-9 1,391 23.236 1,392 -1 96 9.307 1,393 -2 95 -1 1
Benzoic acid, 2-amino-, ethyl ester 87-25-2 1,414 24.091 1,413 1 86 9.662 1,414 0 93 -1 -7
Caryophyllene 87-44-5 1,419 24.340 1,419 0 99 9.751 1,420 -1 99 -1 0
gamma-Elemene 29873-99-2 1,434 24.921 1,433 1 83 9.977 1,434 0 88 -1 -6
Humulene 6753-98-6 1,454 25.707 1,453 1 92 10.293 1,453 1 91 0 1
(E)-beta-Farnesene 18794-84-8 1,457 25.894 1,457 0 96 10.363 1,458 -1 96 -1 0
Alloaromadendrene 25246-27-9 1,461 26.002 1,460 1 92 10.408 1,460 1 84 0 8
gamma-Muurolene 30021-74-0 1,477 26.657 1,476 1 93 10.669 1,476 1 91 0 2
Germacrene D 23986-74-5 1,481 26.824 1,480 1 95 10.736 1,481 0 97 -1 -2
Bicyclogermacrene 24703-35-3 1,496 27.445 1,496 0 98 10.985 1,496 0 97 0 1
alpha-Muurolene 10208-80-7 1,499 27.605 1,500 -1 96 11.048 1,500 -1 93 0 3
alpha-Farnesene 502-61-4 1,508 27.955 1,509 -1 94 11.186 1,509 -1 96 0 -1
γ-Cadinene 39029-41-9 1,513 28.137 1,513 0 97 11.261 1,514 -1 96 -1 1
δ-Cadinene 483-76-1 1,524 28.519 1,523 1 97 11.413 1,524 0 97 -1 1
α-Cadinene 24406-05-1 1,538 29.047 1,537 1 80 11.626 1,537 1 81 0 -2
alpha-Calacorene 21391-99-1 1,542 29.254 1,542 0 94 11.710 1,543 -1 82 -1 13 LR
β-Germacrene 15423-57-1 1,557 29.779 1,556 1 96 11.915 1,556 1 87 0 10 Int
1,6,10-Dodecatrien-3-ol, 3,7,11-trimethyl- 7212-44-4 1,564 30.146 1,565 -1 97 12.091 1,567 -3 97 -2 0
Spathulenol 6750-60-3 1,576 30.574 1,576 0 93 12.244 1,577 -1 85 -1 9
Caryophyllene oxide 1139-30-6 1,581 30.788 1,582 -1 92 12.329 1,583 -2 90 -1 2
tau-Cadinol 5937-11-1 1,640 32.985 1,641 -1 96 13.198 1,641 -1 95 0 2
δ-Cadinol 19435-97-3 1,645 33.163 1,646 -1 92 13.276 1,646 -1 88 0 4
alpha-Cadinol 481-34-5 1,653 33.462 1,654 -1 96 13.396 1,654 -1 94 0 2
Naphthalene, 1,6-dimethyl-4-(1-methylethyl)- 483-78-3 1,674 34.205 1,675 -1 92 13.695 1,674 0 78 1 14 LR, Int
8-Heptadecene 2579-04-6 1,677 34.320 1,678 -1 95 13.731 1,677 0 94 1 2
6,10-Dodecadien-1-ol, 3,7,11-trimethyl- 51411-24-6 1,692 34.795 1,691 1 81 13.928 1,690 2 87 1 -6
2,6,10-Dodecatrien-1-ol, 3,7,11-trimethyl-, (Z,E)- 3790-71-4 1,697 35.110 1,700 -3 92 14.058 1,699 -2 89 1 3
trans-Farnesol 106-28-5 1,722 35.968 1,724 -2 97 14.418 1,724 -2 98 0 -1
Farnesol, 2E, 6Z- 3879-60-5 1,742 36.652 1,743 -1 79 14.676 1,743 -1 78 0 1
all-trans-Farnesyl acetate 4128-17-0 1,843 40.112 1,842 1 94 16.057 1,843 0 92 -1 3
Cubitene 66723-19-1 1,878 41.166 1,874 4 82 16.479 1,874 4 78 0 3
m-Camphorene 20016-73-3 1,960 43.728 1,952 8 95 17.508 1,953 7 93 -1 3
p-Camphorene 20016-72-2 1,995 44.815 1,986 9 92 17.942 1,986 9 91 0 1
Hexadecanoic acid, ethyl ester 628-97-7 1,993 45.083 1,995 -2 73 18.054 1,995 -2 75 0 -2
118
19
Conclusion
Using the techniques described in this application note, a
typical method for the qualitative analysis of essential oils
was successfully converted to a method using hydrogen
carrier gas. The resulting hydrogen method retains the same
chromatographic resolution and relative elution order of the
original method, but with a run time 2.5 times shorter. The
column capacity with the new method is reduced, being a
calculated 36% of the original method, so the amount injected
may need adjustment in some cases.
The new hydrogen method was then applied to the
analysis of two essential oils and found to generate results
comparable to those from the helium method. The use of
spectral deconvolution and retention index search filtering
with Agilent MassHunter Unknowns Analysis gave improved
search results that were faster than previous identification
methods. The NIST23 library, with expanded content for
essential oil components and RIs, allowed identification of a
significant portion of the compounds present.
The Agilent HydroInert source is a key component in the
successful conversion to hydrogen. Without it, in-source
reactions were shown to degrade the spectra of some
compounds to the point where they were misidentified.
References
1. Agilent EI GC/MS Instrument Helium to Hydrogen
Carrier Gas Conversion. Agilent Technologies user guide,
publication number 5994-2312EN, 2020.
2. Blumberg, L. M. Method Translation in Gas
Chromatography. US Patent US6634211B1. 2002.
3. Blumberg, L. M.; Klee, M. S. Method Translation and
Retention Time Locking in Partition GC. Anal. Chem. 1998,
70(18), 3828–3839.
4. Agilent GC Calculators and Method Translation Software.
Tools available for download from: https://www.agilent.
com/en/support/gas-chromatography/gccalculators
5. Agilent Inert Plus GC/MS System with HydroInert Source
Applying H2
Carrier Gas to Real-World GC/MS Analyses.
Agilent Technologies technical overview, publication
number 5994-4889EN, 2022.
6. Godina, L. Flavor and Fragrance GC/MS Analysis with
Hydrogen Carrier Gas and the Agilent HydroInert Source,
Agilent Technologies application note, publication number
5994-6015EN, 2023.
7. MassHunter Unknowns Analysis Video: https://www.
youtube.com/watch?v=y_zJkBfnN3g
8. Adams, R. Identification of Essential Oil Components
by Gas Chromatography/Mass Spectrometry, version 4,
available through Diablo Analytical (Antioch, CA).
9. Sparkman, O. D. NIST 23: The Largest Increases in
Compound Coverage for the Tandem and NIST/EPA/
NIH EI Libraries Since NIST Became Curator, Separation
Science, July 2023. NIST 23: The Largest Increases in
Compound Coverage for the Tandem and NIST/EPA/
NIH EI Libraries Since NIST Became Curator - Separation
Science (sepscience.com)
10. David, F.; Scanlan, F.; Sandra, P.; Szelewski, M. Analysis
of Essential Oil Compounds Using Retention Time
Locked Methods and Retention Time Databases,
Agilent Technologies application note, publication number
5988-6530EN, 2002.
119
20
Appendix
Setting up MassHunter Unknowns Analysis
This section shows how to set up Agilent MassHunter
Unknowns Analysis. The parameters shown here were used
in this specific application. For other applications, different
parameters can be used to optimize the process.
Locate the Agilent MassHunter Quantitative Analysis software
folder under the Microsoft Windows Start menu and open the
MassHunter Unknowns Analysis program.
1. Click File > New Analysis. Navigate to the directory
containing your data files.
2. Enter a file name for the analysis.
3. Click File > Add Samples. Select the data files that
you want to process. You should see the TIC of your
chromatogram appear.
4. Click Method > Edit. Set the parameters as shown in
Appendix Figures 1 to 5.
Leave these
at defaults
Leave these at defaults
Set these
Appendix Figure 1. Setting the parameters for the Peak Detection and Deconvolution tabs in Agilent MassHunter Unknowns Analysis.
120
21
Leave these
at defaults
Set these values
Select NIST23.L
Select the .rtc file created in Notepad
Appendix Figure 2. Setting the parameters for the Library Search tab in
Agilent MassHunter Unknowns Analysis.
Leave these
at defaults
for now
Set to 70
Appendix Figure 3. Setting the parameters for the Compound Identification
tab in Agilent MassHunter Unknowns Analysis.
Leave everything at defaults
Appendix Figure 4. Setting the parameters for the Target Match tab in
Agilent MassHunter Unknowns Analysis.
Leave these at defaults for now
Select Apply to Selected Sample, then Close.
After closing, go to the Analyze menu and select Analyze Sample.
Note: it will take a while to process.
Uncheck this
Appendix Figure 5. Setting the parameters for the Blank Subtraction tab in
Agilent MassHunter Unknowns Analysis.
121
22
Appendix Figure 6. Example result of a deconvoluted/searched data file in Agilent MassHunter Unknowns Analysis.
Right-click the title bar and select Add/Remove Columns.
Select these columns for now.
Library spectrum
More examples of in-source reactions eliminated with
the HydroInert source
In this section, the spectra of several compounds from
the Brazilian orange and neroli oils are examined. For each
compound, the spectrum obtained using helium and the
3 mm inert extractor source is presented with the spectrum
for each compound obtained using hydrogen with the 9 mm
HydroInert source, and the inert extractor source with the
9 and 3 mm extractor lenses. As shown in Figures 7 to 11,
changes in the hydrogen spectra without the HydroInert
source are clear, resulting in reduced LMS values and reduced
confidence in identification.
122
23
Appendix Figure 7. Linalool in Brazilian orange oil. Red arrows indicate spectral changes observed with hydrogen carrier and non-HydroInert sources.
3 mm Inert extractor, He
LMS = 96.7
9 mm HydroInert, H2
LMS = 95.3
Component RT: 11.0513
40 50 60 70 80 90 100 110 120 130 140 150 160
Counts
-1.2
-1.0
-0.8
-0.6
-0.4
-0.2
0
0.2
0.4
0.6
0.8
71.0
93.0
93.0
71.0
43.0 55.0
41.0 55.0
80.0
80.0 121.0
121.0
109.0 136.0
107.0 136.0
150.0
154.0
Component RT: 4.4621
40 50 60 70 80 90 100 110 120 130 140 150 160 170
170
Counts
-1.0
-0.8
-0.6
-0.4
-0.2
0
0.2
0.4
0.6
0.8
1.0
1.0
71.0
93.1
71.0
93.0
43.0 55.0
41.1 55.1
80.1
80.0
121.1
121.0
105.1
136.0
136.1
107.0
149.9
154.0
166.9
Component RT: 4.4841
40 50 60 70 80 90 100 110 120 130 140 150 160 170
Counts
-1.2
-1.0
-0.8
-0.6
-0.4
-0.2
0
0.2
0.4
0.6
0.8
69.1
71.0
41.1 93.1
41.0 93.0
55.0
55.0 79.1 121.1
80.0 121.0
105.1 136.1
107.0 136.0
151.9
9 mm Inert extractor, H2
LMS = 89.5
Component RT: 4.4812
40 50 60 70 80 90 100 110 120 130 140 150 160 170
Counts
-1.2
-1.0
-0.8
-0.6
-0.4
-0.2
0
0.2
0.4
0.6
0.8
1.0
1.0
71.0
69.1 93.1
93.0
41.1
43.0 55.0
55.1 79.1 121.1
80.0 121.0
105.1 136.2
107.0 136.0 154.0
154.0
3 mm Inert extractor, H2
LMS = 89.7
Brazilian orange: Linalool (CAS 78-70-6)
×102
×102
×102
×102
Mass-to-charge (m/z) Mass-to-charge (m/z)
Appendix Figure 8. cis-Carveol in Brazilian orange oil. Red arrows indicate spectral changes observed with hydrogen carrier and non-HydroInert sources. Counts Counts Counts Counts
×102
×102
×102
×102
Mass-to-charge (m/z) Mass-to-charge (m/z)
Component RT: 16.4233
30 40 50 60 70 80 90 100 110 120 130 140 150 160 170
84.0
84.1
109.1 134.1
91.0
41.0 109.0 55.0 134.0
55.1 69.0
69.0
41.1
93.0 119.0
152.0
152.1
Component RT: 6.6160
30 40 50 60 70 80 90 100 110 120 130 140 150 160 170
84.0
84.0
109.0
41.0
91.0 119.0
109.0
134.0
55.0
69.0 134.0
55.0 69.0 41.0
91.0 119.0
152.0
152.0
Component RT: 6.6374
40 50 60 70 80 90 100 110 120 130 140 150 160 170
-1.2
-1.0
-0.8
-0.6
-0.4
-0.2
0
0
0.2
0.4
0.6
0.8
1.0
84.0
91.1 119.1
134.0
134.1
41.0 79.0 109.0
79.1
55.0 91.0
67.1 109.1 41.1
69.0
55.0
152.0
151.9
Component RT: 6.6350
40 50 60 70 80 90 100 110 120 130 140 150 160 170
-1.2
-1.0
-0.8
-0.6
-0.4
-0.2
0.2
0.4
0.6
0.8
1.0
-1.2
-1.0
-0.8
-0.6
-0.4
-0.2
0
0
0.2
0.4
0.6
0.8
1.0
-1.2
-1.0
-0.8
-0.6
-0.4
-0.2
0.2
0.4
0.6
0.8
1.0
84.0
119.0
91.0
134.0
134.0
41.0
84.0
79.0 109.0
109.0
55.0 91.0
67.0
69.0
41.0 55.0
152.0
152.0
9 mm Inert extractor, H2
LMS = 88.0
3 mm Inert extractor, H2
LMS = 90.0
Brazilian orange: cis-Carveol (CAS 1197-06-4)
3 mm Inert extractor, He
LMS = 95.8
9 mm HydroInert, H2
LMS = 96.7
123
24
Appendix Figure 9. trans-Carveol in Brazilian orange oil. Red arrows indicate spectral changes observed with hydrogen carrier and non-HydroInert sources. Counts Counts Counts Counts
×102
×102
×102
×102
Mass-to-charge (m/z) Mass-to-charge (m/z)
Component RT: 15.9464
Component RT: 6.4486 Component RT: 6.4268
Component RT: 6.4461
9 mm Inert extractor, H2
LMS = 84.4
3 mm Inert extractor, H2
LMS = 87.6
Brazilian orange: trans-Carveol (CAS 1197-07-5)
3 mm Inert extractor, He
LMS = 94.8
9 mm HydroInert, H2
LMS = 96.0
40 50 60 70 80 90 100 110 120 130 140 150 160
109.0
109.0
84.0
41.0 55.0
84.0 119.0
91.0 69.0
55.0
77.0
134.0
119.0
41.0 69.0 77.0
137.0
152.0
152.0
40 50 60 70 80 90 100 110 120 130 140 150 160
109.0
109.1
84.0 41.0
84.0
55.0
69.0 91.0
119.1
41.1 55.1 69.1
119.0
137.1
137.0 152.0
151.9
40 50 60 70 80 90 100 110 120 130 140 150 160
-1.2
-1.0
-0.8
-0.6
-0.4
-0.2
0
0.2
0.4
0.6
0.8
1.0
109.0
119.0
91.0
84.0
134.0
41.0
84.0
55.0 91.0 119.0 152.0
67.0
69.0
41.0 55.0
134.0
152.0
40 50 60 70 80 90 100 110 120 130 140 150 160
-1.2
-1.0
-0.8
-0.6
-0.4
-0.2
0
0.2
0.4
0.6
0.8
1.0
-1.2
-1.0
-0.8
-0.6
-0.4
-0.2
0
0.2
0.4
0.6
0.8
1.0
-1.2
-1.0
-0.8
-0.6
-0.4
-0.2
0
0.2
0.4
0.6
0.8
1.0
91.1
109.0
119.1
84.0
109.1
134.1
41.0 55.0
77.1
91.0 119.0
67.1
69.0 152.0
41.1
134.0
55.0
151.9
Appendix Figure 10. p-Cymen-8-ol in neroli oil. Red arrows indicate spectral changes observed with hydrogen carrier and non-HydroInert sources.
3 mm Inert extractor, He
LMS = 91.0
9 mm HydroInert, H2
LMS = 89.6
Component RT:
14.5145
40 50 60 70 80 90 100 110 120 130 140 150 160
Counts
-1.2
-1.2
-1.0
-0.8
-0.6
-0.4
-0.2
0
0.2
0.4
0.6
0.8
Component RT: 5.8637
40 50 60 70 80 90 100 110 120 130 140 150 160 170 40 50 60 70 80 90 100 110 120 130 140 150 160 170
170
Counts
-1.0
-0.8
-0.6
-0.4
-0.2
0
0.2
0.4
0.6
0.8
1.0
1.0
Component RT: 5.8893
Counts
-1.2
-1.0
-0.8
-0.6
-0.4
-0.2
0
0.2
0.4
0.6
0.8
9 mm Inert extractor, H2
LMS = 75.4
Component RT: 5.8907
40 50 60 70 80 90 100 110 120 130 140 150 160 170
Counts
-1.2
-1.0
-0.8
-0.6
-0.4
-0.2
0
0.2
0.4
0.6
0.8
1.0
1.0
3 mm Inert extractor, H2
LMS = 79.8
Neroli oil: p-Cymen-8-ol (CAS 1197-01-9)
×102
×102
×102
×102
Mass-to-charge (m/z) Mass-to-charge (m/z)
43.0
132.0 117.0
43.0
135.0
91.0
91.0
65.0
65.0 117.0 51.0 77.0
77.0 150.0
105.0
102.0
150.0
43.0
135.0
43.1
117.0
135.0
91.1
91.0
65.1
65.0 117.0
77.0 105.1 150.1
39.0 77.0
51.1
51.0 105.0 150.0
43.0
43.1
134.9
91.1
135.0
116.9
91.0
64.9 77.1
65.0 117.0
50.9 149.9
51.0 77.0 105.0
101.9
150.0
167.9
43.0
117.0 132.0
43.0
91.0
135.0
91.0
65.0
65.0 117.0
51.0 77.0 105.0
77.0
150.0
51.0 105.0 150.0
124
www.agilent.com
DE52560836
This information is subject to change without notice.
© Agilent Technologies, Inc. 2024
Printed in the USA, January 26, 2024
5994-7058EN
Appendix Figure 11. (3E,5E)-2,6-Dimethylocta-3,5,7-trien-2-ol in neroli oil. Red arrows indicate spectral changes observed with hydrogen carrier and
non-HydroInert sources.
3 mm Inert extractor, He
LMS = 88.1
9 mm HydroInert, H2
LMS = 88.9
40 50 60 70 80 90 100 110 120 130 140 150 160
Counts
-1.2
-1.2
-1.0
-0.8
-0.6
-0.4
-0.2
0
0.2
0.4
0.6
0.8
40 50 60 70 80 90 100 110 120 130 140 150 160 170 40 50 60 70 80 90 100 110 120 130 140 150 160 170
170
Counts
-1.0
-0.8
-0.6
-0.4
-0.2
0
0.2
0.4
0.6
0.8
1.0
1.0
Component RT: 6.2480
Component RT: 6.2447
Counts
-1.2
-1.0
-0.8
-0.6
-0.4
-0.2
0
0.2
0.4
0.6
0.8
9 mm Inert extractor, H2
LMS = 77.3
40 50 60 70 80 90 100 110 120 130 140 150 160 170
Counts
-1.2
-1.0
-0.8
-0.6
-0.4
-0.2
0
0.2
0.4
0.6
0.8
1.0
1.0
3 mm Inert extractor, H2
LMS = 75.6
Neroli oil: (3E,5E)-2,6-Dimethylocta-3,5,7-trien-2-ol (CAS 206115-88-0)
×102
×102
×102
×102
Mass-to-charge (m/z) Mass-to-charge (m/z)
Component RT: 15.4812
43.0
91.0
91.0 109.0
119.0
43.0
81.0 119.0
79.0
67.0
134.0
55.0 152.0
152.0
134.0
55.0 67.0
Component RT: 6.2287
43.0
91.0
91.0 109.0
79.0
81.0 119.0
43.0
67.0 55.0 152.0
109.0 55.0 67.0
134.0
134.0 152.0 72.0
43.0
93.0
121.0
77.0
91.0 109.0
81.0 119.0
109.0 136.0
67.0
41.0 67.0
55.0 152.0 134.0
55.0
152.0 72.0
43.0
93.0
109.0
121.0
91.0
81.0
77.0
119.0
105.0 136.0
67.0 55.0 152.0 134.0
41.0 53.0 67.0 84.0 152.0
Application Note
Food Testing
Authors
Praveen Arya, Soma Dasgupta,
and Vivek Dhyani
Agilent Technologies, Inc.
Abstract
This application note demonstrates the use of an Agilent 8890 GC system coupled
with an Agilent 7010 GC/MS triple quadrupole mass spectrometry system to
detect and quantify ethylene oxide (EtO) and 2-chloroethanol (2-CE) simultaneously
in foodstuffs such as flaxseed, cumin powder, and red chili powder. Sample
preparation was done using QuEChERS extraction and a dispersive cleanup process
followed by injection into GC/MS/MS through liquid injection. A limit of quantification
(LOQ) of 10 ppb for both compounds was achieved in matrix. Average recoveries
ranged from 75 to 86% for both compounds.
Estimation of Ethylene Oxide and
2-Chloroethanol in Spices and
Oilseeds Using QuEChERS Extraction
and GC/MS/MS
Agilent 8890/7010 triple quadrupole GC/MS system
126 Return to Table of Contents
2
Introduction
EtO is used to sterilize foods to eliminate insects and bacteria,
such as salmonella. Ethylene chlorohydrin, or 2-CE, is a
derivative produced by the reaction of EtO with chlorine ions
present in foodstuff. Use of EtO is banned in the European
Union (EU) due to its carcinogenic and toxic properties.
Previously, methods for analysis of EtO (EtO and 2-CE) have
been developed that include acidic conversion of EtO to 2-CE.
These methods are time-consuming, labor-intensive, and
require large quantities of harmful solvents. Due to the volatile
nature of EtO, sample preparation is crucial. In December
2020, the EU Reference Laboratories (EURL) for Residues of
Pesticides recommended a single-residue method for the
analysis of EtO and 2-CE in sesame seeds using QuEChERS
extraction followed by GC/MS/MS analysis.
The method adopted in this work demonstrates the use of an
automated liquid sampler for sample introduction to the 8890
GC system coupled with a 7010 GC/MS triple quadrupole
mass spectrometry system.
Parameter Value
GC/MS/MS Method Parameters
GC Agilent 8890 GC with G4513 autosampler
Mass Spectrometer Agilent 7010 triple quadrupole mass spectrometry system
Analytical Column Agilent J&W DB-VRX (60 m x 0.25 mm, 1.4 µm)
Column Flow Helium: 1.0 mL/min, constant flow
Injection Mode Pulsed split (4:1)
Injection Volume 2 µL
Injection Program Starts at 90 °C (hold for 0.8 min), ramped at 450 °C/min to
250 °C, hold for 10 min
Oven Program Starts at 40 °C (hold for 1.0 min), ramped at 10 °C/min to
160 °C, and then at 30 °C/min to 245 °C, hold for 5 min
MS Parameters Ionization mode: EI; Ion source temperature: 230 °C;
Quadrupole temperature (Q1 and Q2): 150 °C
MRM Transitions
EtO
44 & 14 (CE:20)
44 & 28 (CE:5)
44 & 29 (CE:5)
2-CE
80 & 31 (CE:5)
80 & 43 (CE:5)
82 & 31 (CE:5)
Table 1. GC/TQ parameters.
Experimental
Standard preparation
Due to the high volatility of EtO, its standard solutions
were prepared at a low temperature (< 10 °C). As a diluent,
acetonitrile was placed in a freezer (at –20 °C) for at
least 15 minutes before use. The cold analytical standard
solutions were diluted with cold acetonitrile to prepare the
stock standard solutions of EtO and 2-CE, which had a
concentration of 1 mg/mL each.
The stock standard solution was further diluted with
acetonitrile to obtain a working standard solution which had a
concentration of 10 µg/ml for EtO and 2-CE. All the calibration
standards (2.0, 5.0, 10.0, 20.0, 50.0, 100, and 200 ng/mL)
were freshly prepared by diluting the stock solution with
acetonitrile. Matrix-matched standards of flaxseeds, cumin
powder, and red chili powder were prepared by post-spiking
the requisite amount of solution to extracts of each matrix.
Before analysis, all stock solutions were preserved at a
temperature of −20 °C to avoid degradation.
Sample preparation
Homogenized samples of flaxseed, cumin powder, and red
chili powder were processed using the QuEChERS extraction
procedure according to the EN 15662 procedure (Figure 1).
Approximately 2.00 ± 0 .01 g of each sample was weighed
in 50 mL centrifuge tubes. Ten mL of cold water was added
to the centrifuge tubes followed by capping and vortex
mixing for 1 minute to ensure sample hydration, followed by
the addition of 10.0 mL of cold acetonitrile along with two
ceramic homogenizers to improve the extraction efficiency.
The centrifuge tubes were capped and shaken for 10 minutes.
The QuEChERS extraction salt (4 g of MgSO4
, 1 g of NaCl, 1 g
of sodium citrate, and 0.5 g of disodium citrate sesquihydrate)
was added, and the tubes were shaken for another
3 minutes. The samples were then centrifuged for 5 minutes
at 6,000 rpm. The upper acetonitrile layer (6.0 mL) was
transferred to QuEChERS Dispersive Kit 15 mL tubes (150 mg
of PSA, 150 mg of C18EC , and 900 mg MgSO4
). The tubes
were vortexed for 30 seconds followed by centrifugation at
5,000 rpm for 5 minutes. After centrifugation, the supernatant
was transferred into GC vials for analysis.
127
3
Results and discussion
Calibration
Matrix calibrations were performed for EtO and 2-CE at
concentrations of 2, 5, 10, 20, 50, and 100 ng/mL in solvent.
Similar calibrations were performed in post-spike matrix
extracts for flaxseed, cumin powder, and red chili powder.
Excellent values for R2
> 0.99 were obtained.
Figures 8 and 9 show linearity of EtO and 2-CE respectively in
acetonitrile. Figures 10 and 11 show linearity of EtO and 2-CE
respectively in a flaxseed matrix.
Figure 1. QuEChERS workflow for the extraction and cleanup of samples.
Standard area repeatability
A repeatable elution was obtained by injecting a 10 ppb
concentration of EtO and 2-CE in a matrix extract. As shown in
Table 2, a % RSD data of EtO and 2-CE is calculated from peak
areas of 6 replicate injections of 10 ppb matrix standards in
flaxseed, cumin powder, and red chili powder.
Recovery
EtO and 2-CE were spiked in samples of flaxseed, cumin
powder, and red chili powder at concentration levels of
20 and 50 ng/g. Acceptable recoveries were obtained by
quantification through post-spike matrix-based calibration.
Results are highlighted in Table 3.
Transfer 6.00 mL of supernatant to a 15 mL dSPE tube.
Cleanup Step
Agitate for 30 seconds.
Centrifuge at 5,000 rpm for 5 minutes.
Transfer the clear supernatant to a 2 mL vial for injection.
Weigh 2.00 g ± 0.01 g of sample into a 50 mL centrifuge tube.
QuEChERS Extraction Step
Add water and shake briefly to wet the sample.
Add 10.00 mL of ACN and extraction aids and mix for 15 minutes.
Add water and shake briefly to wet the sample.
Add extraction salts and mix for 3 minutes.
Centrifuge at 6,000 rpm for 5 minutes.
128
4
A B
EtO
2-CE
Figure 3. Overlaid chromatograms from a set of calibration standards from 2 ng/mL to 100 ng/mL; (A) EtO and (B) 2-CE.
Figure 2. Chromatograms for (A) EtO at the 10 ng/mL level and (B) 2-CE at the 10 ng/mL level.
A
B
EtO
2-CE
129
5
Figure 4. Quantifier and qualifier peaks of EtO at 10 ng/g matrix standard
in flaxseed.
Figure 5. Quantifier and qualifier peaks of 2-CE at 10 ng/g matrix standard
in flaxseed.
Figure 6. Quantifier and qualifier peaks of EtO at 10 ng/g matrix standard
in cumin powder.
Figure 7. Quantifier and qualifier peaks of 2-CE at 10 ng/g matrix standard
in cumin powder.
Figure 8. Calibration curve for EtO in acetonitrile (R2
> 0.998).
Figure 9. Calibration curve for 2-CE in acetonitrile (R2
> 0.998).
Figure 10. Calibration curve for EtO in flaxseed (R2
> 0.999).
Figure 11. Calibration curve for 2-CE in flaxseed (R2
> 0.996).
130
6
Number of
Injections
(Replicates)
EtO (Peak Area) 2-CE (Peak Area)
Flaxseed Cumin Powder Chili Powder Flaxseed Cumin Powder Chili Powder
10 ng/mL
Injection 1 2,266 2,193 3,135 1,065 893 735
Injection 2 2,264 2,205 3,445 1,049 1,035 910
Injection 3 2,545 2,179 3,425 1,129 1,125 880
Injection 4 2,562 2,162 3,440 993 1,048 755
Injection 5 2,392 2,228 3,385 1,236 1,168 845
Injection 6 2,526 2,135 3,335 1,100 979 785
Mean 2,426 2,184 3,361 1,095 1,041 818
SD 138.32 32.76 118 83.04 99.01 70.68
RSD (%) 5.70 1.50 3.51 7.58 9.51 8.64
Table 2. Standard area repeatability for 6 replicates of 10 ppb matrix standard in flaxseed, cumin powder, and red chili powder.
Food Matrix
EtO 2-CE
Spiked
Concentration
(ng/g)
Average Obtained
Concentration
(ng/g)
Recovery
(%)
Spiked
Concentration
(ng/g)
Average Obtained
Concentration
(ng/g)
Recovery
(%)
Flaxseed 20 15.12 75.6 20 15.84 79.2
Cumin Powder 50 39.02 78.0 50 43.29 86.6
Chili Powder 50 38.52 77.04 50 42.47 84.9
Table 3. Percent Recovery of EtO and 2-CE in flaxseed and cumin powder spiked at 20 and 50 ng/g levels, respectively.
Conclusion
An accurate and rugged method was developed which meets
the requirements of the EURL for a single-residue method
that uses QuEChERS extraction followed by GC/MS/MS
analysis for the analysis of EtO and 2-CE in flaxseed, cumin
powder, and red chili powder. The LOQ of the method
was demonstrated at 10 ng/g for all the tested matrices.
Repeatable results are found for 6 successive replicates of
matrix-based standards at 10 ng/g concentration levels for
EtO and 2-CE. Excellent recoveries were obtained for both
EtO and 2-CE in all tested matrices at 20 and 50 ng/g spiked
concentration levels. Thus, this study demonstrates the
usefulness of the developed method for the routine analysis
of food samples for EtO and 2-CE at trace levels.
131
www.agilent.com
DE-000364
This information is subject to change without notice.
© Agilent Technologies, Inc. 2024
Printed in the USA, September 3, 2024
5994-7720EN
References
1. Dunkelberg, H. Carcinogenic Activity of Ethylene
Oxide and Its Reaction Products 2-Chloroethanol,
2-Bromoethanol, Ethylene Glycol, And Diethylene Glycol.
I. Carcinogenicity of Ethylene Oxide in Comparison with
1,2-Propylene Oxide after Subcutaneous Administration
in Mice. Zentralblatt Fur Bakteriologie Mikrobiologie Und
Hygiene. 1. Abt. Originale B Hygiene 1981, 174, 383–404.
(PubMed)
2. Tateo, F.; Bononi, M. Determination of Ethylene
Chlorohydrin as Marker of Spices Fumigation with
Ethylene Oxide. Journal of Food Composition and Analysis
2006, 19, 83–87.
3. Regulation (EU) 2015/868 of 26 May 2015 Amending
Annexes II, III and V to Regulation (EC) No 396/2005
of the European Parliament and of the Council as
Regards Maximum Residue Levels for 2,4,5-T, Barban,
Binapacryl, Bromophos-Ethyl, Camphechlor (Toxaphene),
Chlorbufam, Chloroxuron, Chlozolinate, Dnoc, Di-Allate,
Dinoseb, Dinoterb, Dioxathion, Ethylene Oxide, Fentin
Acetate, Fentin Hydroxide, Flucycloxuron, Flucythrinate,
Formothion, Mecarbam, Methacrifos, Monolinuron,
Phenothrin, Propham, Pyrazophos, Quinalphos,
Resmethrin, Tecnazene and Vinclozolin in or on Certain
Products. Off. J. Eur. Union L. 2015, 145, 1–71.
4. Regulation (EC) No 149/2008 of 29 January 2008
Amending Regulation (EC) No 396/2005 of the European
Parliament and of the Council by Establishing Annexes II,
III and IV Setting Maximum Residue Levels for Products
covered by Annex I thereto. Off. J. Eur. Union L. 2008, 58,
1–398.
5. EURL-SRM - Analytical Observation Report: Analysis of
Ethylene Oxide and Its Metabolite 2-Chloroethanol by
the QuOil or the QuEChERS Method and GC-MS/MS.
December 2020.
Application Note
Flavor and Fragrance
Authors
Yufeng Zhang and
Lay Peng Tan
Agilent Technologies Inc.
Abstract
This application note describes a method for quantitation of four aldehydes
(hexanal, furfural, phenylacetaldehyde, and trans-2-nonenal) responsible for
off-flavors in beer using an Agilent 8890/5977C GC/MSD with an Agilent PAL3
(SPME) autosampler. The method used fully automated, solvent-free extraction
and on-fiber derivatization. The aldehyde compounds were derivatized with the
derivatization agent O-(2,3,4,5,6-pentafluorobenzyl)hydroxylamine hydrochloride
(PFBHA) using the on-fiber derivatization procedure. The derivatization agent was
first adsorbed onto an Agilent 65 µm PDMS/DVB fiber. Next, the fiber was inserted
into a 20 mL headspace vial containing a 2 mL beer sample with agitation at 60 °C
for 30 minutes. Both extraction and derivatization procedures were automatically
performed using the PAL3 autosampler. The method demonstrated excellent
sensitivity, with detection limits of 0.0009 µg/L for hexanal, 0.52 µg/L for furfural,
0.015 µg/L for phenylacetaldehyde, and 0.003 µg/L for trans-2-nonenal. The
limits of the quantification for the four compounds were 0.003, 1.72, 0.05, and
0.01 µg/L, respectively. The four aldehydes were successfully quantified in four beer
samples purchased from a supermarket. Good repeatability was demonstrated
with RSD < 4.9% based on three replicate injections of the four beer samples for all
four aldehydes.
Analysis of Aldehydes in Beer by
Agilent PAL3 Autosampler and
5977C GC/MSD
Return to Table of Contents 133
2
Introduction
Aldehydes are a critical group of compounds in beer that
significantly influence its flavor and aroma profile. These
volatile organic compounds, even at low concentrations,
can impact undesirable sensory characteristics such as
cardboard, grassy, or stale flavors, which negatively affect
the overall quality and consumer acceptance of the product.
The presence of aldehydes is often linked to oxidation
processes during brewing, packaging, and storage, making
them key indicators of beer freshness and stability.1
Hexanal,
furfural, phenylacetaldehyde, and trans-2-nonenal are among
the most recognized aldehydes responsible for off-flavors,
with flavor thresholds of 350 μg/L, 15.157 mg/L, 105 μg/L,
and 0.1 μg/L, respectively.2
Monitoring and controlling aldehyde levels in beer are
essential for maintaining product consistency and ensuring
a high-quality drinking experience. By accurately quantifying
aldehydes, brewers can identify potential issues in their
production processes, such as oxygen exposure or ingredient
degradation, and implement corrective measures to minimize
off-flavors. Additionally, understanding the aldehyde profile of
beer can aid in the development of new brewing techniques
and formulations that enhance flavor stability and shelf life.
Solid-phase microextraction (SPME) is a widely used,
solvent-free sample preparation technique that operates on
the principles of adsorption and desorption. It uses a fiber
coated with an extractive phase to concentrate analytes from
a sample. Various types of fibers are available, including
PDMS, acrylate, carbon WR, DVB, and combinations of these
sorbents, to address the differing polarities of analytes.
SPME is extensively used in a range of analyses, including
environmental characterization, food and flavor analysis,
pharmaceutical studies, and forensics investigations. It is well
suited for automated sample preparation, resulting in reduced
preparation time per sample, minimized risk of human error,
and the freeing up of lab personnel from repetitive tasks.
This application note demonstrates the use of a PAL3 RTC
sampler with SPME tool and 8890/5977C GC/MSD for the
analysis of the four aldehyde compounds in beer. The SPME
sampling tool is equipped with its own read-and-write chip
with preset parameters including the stationary phase and
usage tracking. Both sample extraction and derivatization
were automated with the PAL3 RTC sampler.
Experimental
Reagents and samples
Aldehyde standards (hexanal, furfural, phenylacetaldehyde,
and trans-2-nonenal) and derivatization reagent PFBHA were
purchased from Sigma-Aldrich. HPLC-grade methanol was
from Merck. The water was Milli-Q Ultrapure. Four different
brands of beer were purchased from a local supermarket.
Standards preparation
A mixed stock solution consisting of 10 µg/mL hexanal,
10 µg/mL phenylacetaldehyde, 1,000 µg/mL furfural, and
1 µg/mL trans-2-nonenal was prepared in methanol.
A secondary mixed stock solution consisting of 100 µg/L
hexanal, 100 µg/L phenylacetaldehyde, 10 µg/mL furfural, and
10 µg/L trans-2-nonenal was prepared in ultrapure water.
Using the secondary mixed stock solution, a series of
calibration standards were prepared with a concentration
range of 0.05 to 10 µg/L for hexanal, 5 to 1,000 µg/L for
furfural, 0.1 to 50 µg/L for phenylacetaldehyde, and 0.025 to
5 µg/L for trans-2-nonenal.
Two milliliters of each calibration standard was added to a
20 mL headspace vial and immediately capped for analysis.
PFBHA derivatization reagent with a concentration of
60 mg/L was prepared by weighing 30.1 mg of powder
PFBHA into a 500 mL volumetric flask and dissolving in
ultrapure water up to the 500 mL mark. Ten milliliters of the
60 mg/L PFBHA solution was added to a headspace vial to be
used for sample derivatization.
Sample preparation
Beer samples were stored in a refrigerator at 4 to 6 °C prior
to analysis. A 250 mL portion of the beer was poured into a
clean plastic bottle and capped. The sample was degassed
by hand shaking the capped bottle five times followed
by opening the cap to release the carbon dioxide (CO2
).
The degassing of the samples was performed a total of
three times. A 2 mL degassed beer sample was transferred
to a 20 mL headspace vial, which was immediately capped
for analysis.
134
3
PAL3 RTC and GC/MSD parameters
The analysis parameters of the PAL3 RTC sampler are shown
in Table 1.
Table 1. Agilent PAL3 autosampler and GC/MSD parameters for
beer analysis.
Agilent PAL3 (SPME)
Fiber Type Agilent 65 µm PDMS/DVB (p/n 5610-5873)
Fiber Conditioning Temperature 250 °C
Preconditioning Time 5 min
Incubation Time 20 min
Incubation Temperature 60 °C
Derivatization Time 10 min
Agitator Speed 250 rpm
Sample Extraction Time 30 min
Sample Desorption Time 1 min
Post Conditioning Time 5 min
Gas Chromatograph
Model Agilent 8890 GC
GC Column Agilent J&W DB-5ms UI, 30 m × 0.25 mm,
0.25 µm (p/n 122-5532UI)
Column Pneumatics Constant flow
Carrier Gas Helium
Injector Mode Splitless
Purge Flow to Split Vent 50 mL/min at 2 min
Inlet Temperature 250 °C
Injector Liner Agilent Ultra Inert splitless liner
(p/n 5190-4047)
Flow Rate 1.2 mL/min
Oven Temperature Program
60 °C for 2 min
10 °C/min to 140 °C
7 °C/min to 250 °C, hold 3.0 min
Equilibration Time 3 min
Mass Spectrometer
Model Agilent 5977C GC/MSD
Ionization Mode EI, 70eV
Acquisition Mode Scan
Scan Speed N = 2
Scan Range 50 to 520 amu
GC Transfer Line Temperature 250 °C
Ion Source Temperature 230 °C
Quad Temperature 150 °C
Results and discussion
Compound identification and retention time confirmation
The 10 μg/L calibration standard was analyzed in full scan
data acquisition mode, and the total ion chromatogram (TIC)
is shown in Figure 1.
Figure 1. TIC of 10 µg/L aldehydes with Agilent SPME on-fiber
PFBHA derivatization.
The data file for the 10 µg/L sample was processed using
MassHunter Unknowns Analysis software. Automatic
deconvolution of the data was performed using Unknowns
Analysis to identify the components present exclusively in
the sample. From the resulting list of components, the four
target aldehydes were identified through library matching
against the NIST 23 spectral library, achieving match scores
above 80. The retention times (RTs) of the four aldehyde
derivatives were identified as 13.299, 13.830, 17.419, and
18.914 minutes, respectively (Figures 2 to 5).
135
4
Figure 2. Hexanal derivative identified with an RT of 13.299 minutes.
Figure 3. Furfural derivative identified with an RT of 13.830 minutes.
136
5
Figure 4. Phenylacetaldehyde derivative identified with an RT of 17.419 minutes.
Figure 5. trans-2-Nonenal derivative identified with an RT of 18.914 minutes.
137
6
Calibration curves
Based on the response of the calibration standard solutions,
the calibration curves were plotted for the four aldehyde
derivatives. The results are shown in Table 2 and Figures 6
to 9.
Table 2. The calibration range and R2
for the four aldehydes.
No. Compound Name Calibration Range (µg/L) R2
1 Hexanal 0.05 to 10 0.999
2 Furfural 5 to 1,000 0.998
3 Phenylacetaldehyde 0.1 to 50 0.996
4 trans-2-Nonenal 0.025 to 5 0.998
Figure 6. Calibration curve for hexanal 0.05 to 10 μg/L.
Hexanal
0 1 2 3 4 5 6 7 8 9 10
Responses
0
0.2
0.4
0.6
0.8 y = 1,019,452.066254x + 31,382.352344
R2
= 0.9992
R = 0.9997
×107
Concentration (µg/L)
Figure 7. Calibration curve for furfural 5 to 1,000 μg/L. Responses
0
0.2
0.4
0.6
0.8
×107
Concentration (µg/L)
Furfural
0 100 200 300 400 500 600 700 800 900 1,000
y = 10,983.666729x – 17,133.067454
R2
= 0.9980
R = 0.9989
Figure 8. Calibration curve for phenylacetaldehyde 0.1 to 50 μg/L. Responses ×106
Concentration (µg/L)
Phenylacetaldehyde
0 5 10 15 20 25 30 35 40 45 50
0
1
2
3
4
5
6 y = 140,635.827993x – 5,702.613289
R2
= 0.9963
R = 0.99970
Figure 9. Calibration curve for trans-2-nonenal 0.025 to 5 μg/L. Responses ×106
Concentration (µg/L)
0
trans-2-Nonenal
0 0.5 1.0 1.5 2.0 2.5 3.0 3.5 4.0 4.5 5.0
0.5
1.0
1.5 y = 22,317.224704x2
+ 233,186.59012 x – 5,014.216502
R2
= 0.9980
R = 0.9979
Type: Quadratic, Origin:Ignore,
Weight:1/x
138
7
Quantification results of beer samples
Based on the calibration curve setup, quantification was
performed for the four aldehydes in the four brands of beer
samples that were tested. The quantification results are
summarized in Tables 3 to 6. All the aldehydes in the four beer
samples were below their respective flavor thresholds. Among
the four brands of beer samples that were tested, Brand 4
contained the lowest levels of aldehydes, with 0.45 μg/L
hexanal, 6.26 μg/L furfural, 6.64 μg/L phenylacetaldehyde,
and 0.031 μg/L trans-2-nonenal.
Table 3. Quantification results of hexanal in four brands of beer samples.
No. Beer
Hexanal
Concentration (µg/L)
Average
Concentration
(µg/L)
Concentration
%RSD (n = 3)
1 Brand 1 0.67 0.67 0.69 0.68 1.7
2 Brand 2 1.53 1.54 1.61 1.56 2.8
3 Brand 3 0.99 1.03 1.04 1.02 2.6
4 Brand 4 0.45 0.46 0.45 0.45 1.3
Table 4. Quantification results of furfural in four brands of beer samples.
No. Beer
Furfural
Concentration (µg/L)
Average
Concentration
(µg/L)
Concentration
%RSD (n = 3)
1 Brand 1 18.45 18.75 18.45 18.55 0.9
2 Brand 2 49.46 49.39 52.27 50.37 3.3
3 Brand 3 24.81 26.53 25.04 25.46 3.7
4 Brand 4 6.10 6.37 6.30 6.26 2.2
Table 5. Quantification results of phenylacetaldehyde in four brands of
beer samples.
No. Beer
Phenylacetaldehyde
Concentration (µg/L)
Average
Concentration
(µg/L)
Concentration
%RSD (n = 3)
1 Brand 1 11.07 10.41 10.05 10.51 4.9
2 Brand 2 8.36 8.26 8.07 8.23 1.8
3 Brand 3 8.72 8.63 8.92 8.76 1.7
4 Brand 4 6.84 6.59 6.48 6.64 2.8
Table 6. Quantification results of trans-2-nonenal in four brands of
beer samples.
No. Beer
Trans-2-Nonenal
Concentration (µg/L)
Average
Concentration
(µg/L)
Concentration
%RSD (n = 3)
1 Brand 1 0.034 0.035 0.034 0.034 1.7
2 Brand 2 0.061 0.063 0.063 0.062 2.8
3 Brand 3 0.034 0.034 0.035 0.034 1.7
4 Brand 4 0.030 0.031 0.031 0.031 1.3
139
8
With three replicate injections for each beer sample, the
concentration %RSDs were calculated to be below 4.9%. The
TIC overlays of the three replicate injections are shown in
Figures 10 to 13.
Figure 10. TIC overlay of the four aldehydes in the Brand 1 beer sample.
1
2
3
4
5
×105
13.2 13.3 13.4
×105
13.7 13.8 13.9 14.0
0.2
0.4
0.6
0.8
1.0
0.2
0.4
0.6
0.8
1.0
1.2
1.4
1.6
1.8
Hexanal
×106
17.3 17.4 17.5
Phenylacetaldehyde
Furfural
×103
18.8 18.9 19.0 19.1
0.2
0.4
0.6
0.8
1.0
1.2
1.4
1.6
trans-2-Nonenal
Figure 11. TIC overlay of the four aldehydes in the Brand 2 beer sample.
×106
13.2 13.3 13.4
×105
13.7 13.8 13.9 14.0
0.2
0.4
0.6
0.8
1.0
1.2
Hexanal
×105
17.3 17.4 17.5
Phenylacetaldehyde
Furfural
×103
18.8 18.9 19.0 19.1
trans-2-Nonenal
0.5
1.0
1.5
2.0
2.5
3.0
3.5
0.5
1.0
1.5
2.0
2.5
3.0
3.5
4.0
4.5
1
2
3
4
5
6
7
8
140
9
Figure 12. TIC overlay of the four aldehydes in the Brand 3 beer sample.
×105
13.2 13.3 13.4
×105
13.7 13.8 13.9 14.0
Hexanal
×106
17.3 17.4 17.5
Phenylacetaldehyde
Furfural
×103
18.8 18.9 19.0 19.1
trans-2-Nonenal
1
2
3
4
5
6
7
0.2
0.4
0.6
0.8
1.0
1.2
1.4
0.2
0.4
0.6
0.8
1.0
1.2
1.4
1.6
1.8
0.1
0.2
0.3
0.4
0.5
0.6
0.7
0.8
Figure 13. TIC overlay of the four aldehydes in the Brand 4 beer sample.
×105
13.2 13.3 13.4
×105
13.7 13.8 13.9 14.0
Hexanal
×105
17.3 17.4 17.5 17.6
Phenylacetaldehyde
Furfural
×103
18.8 18.9 19.0 19.1
trans-2-Nonenal
0.2
0.4
0.6
0.8
1.0
1.2
0.2
0.4
0.6
0.8
1.0
0.5
1.0
1.5
2.0
2.5
3.0
3.5
1
2
3
4
5
6
7
141
www.agilent.com/chem/5977c
DE58984949
This information is subject to change without notice.
© Agilent Technologies, Inc. 2024
Printed in the USA, July 18, 2024
5994-7633EN
Determination of detection limits
Using the lowest calibration concentration of each compound,
the signal-to-noise ratio (S/N) in full scan mode was
calculated. The limit of quantification (LOQ) was determined
at a S/N of 10, and the limit of detection (LOD) was
determined at a S/N of 3. A summary of the LOQ and LOD
results is presented in Table 7.
Table 7. LOQ and LOD of the aldehydes.
Aldehyde S/N LOQ (µg/L) LOD (µg/L)
Hexanal (0.05 µg/L) 161 0.003 0.0009
Furfural (5 µg/L) 29 1.72 0.52
Phenylacetaldehyde (0.1 µg/L) 20 0.05 0.015
trans-2-Nonenal (0.025 µg/L) 24 0.01 0.003
Conclusion
This application note describes the quantitative analysis
of four aldehydes (hexanal, furfural, phenylacetaldehyde,
and trans-2-nonenal) responsible for off-flavors in beer
using an Agilent 8890/5977C GC/MSD with a PAL3 (SPME)
autosampler. This method offers the advantages of full
automation, rapid analysis, solvent-free extraction, and
on-fiber derivatization. With this automated solution, excellent
sensitivity was demonstrated for the detection of hexanal
(0.0009 μg/L), furfural (0.52 μg/L), phenylacetaldehyde
(0.015 μg/L), and trans-2-nonenal (0.003 μg/L). Four different
brands of beer were analyzed, with hexanal detected in the
range of 0.45 to 1.56 μg/L, furfural in the range of 6.62 to
50.37 μg/L, phenylacetaldehyde in the range of 6.64 to
10.51 μg/L, and trans-2-nonenal in the range of 0.031 to
0.062 μg/L. Good repeatability was demonstrated with
RSD < 4.9% based on three replicate injections of the four
beer samples for all four aldehydes.
References
1. Aguiar, D.; et al. Assessment of Staling Aldehydes in Lager
Beer under Maritime Transport and Storage Conditions.
Molecules 2022, 27(3), 600.
2. Moreira, M. T. G.; et al. Aldehyde Accumulation in Aged
Alcoholic Beer: Addressing Acetaldehyde Impacts on
Upper Aerodigestive Tract Cancer Risks. Int. J. Mol. Sci.
2022, 23(22), 14147.
DE-002126
This information is subject to change without notice.
© Agilent Technologies, Inc. 2024
Published in the USA, November 19, 2024
5994-7858EN
Learn more:
www.agilent.com/chem/5977c
www.agilent.com/chem/7000e
www.agilent.com/chem/7010
Buy online:
www.agilent.com/chem/store
Find a local Agilent customer center in your country:
www.agilent.com/chem/contactus
U.S. and Canada
1-800-227-9770
agilent_inquiries@agilent.com
Europe
info_agilent@agilent.com
Asia Pacific
inquiry_lsca@agilent.com
Brought to you by
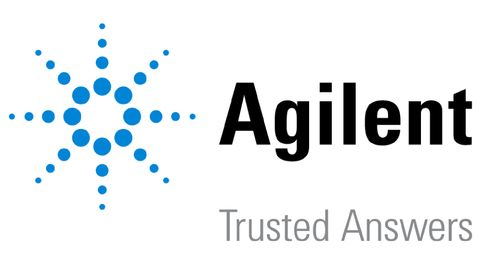
Download the Compendium for FREE Now!
Information you provide will be shared with the sponsors for this content. Technology Networks or its sponsors may contact you to offer you content or products based on your interest in this topic. You may opt-out at any time.
Experiencing issues viewing the form? Click here to access an alternate version