The Dawning of Multimodal Genetics
The genomics revolution fell short of its promises, but new technology may get us there.
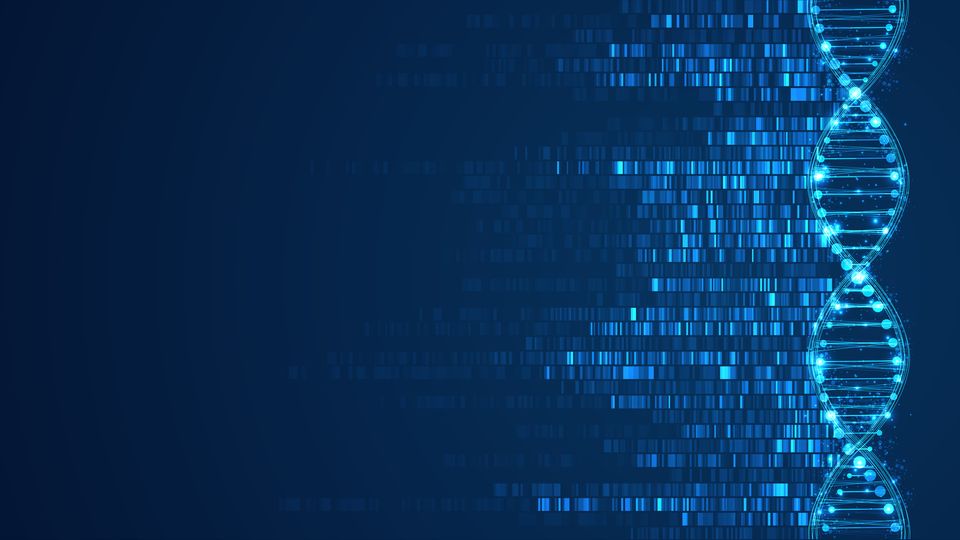
Complete the form below to unlock access to ALL audio articles.
DNA is often called the blueprint of life – a molecular code thought to dictate everything from eye color to disease risk. With the completion of the Human Genome Project in 2003, many expected the secrets of conditions like cancer and heart disease to unravel, ushering in an era of breakthrough treatments and genome-guided precision medicine.
Now more than 20 years later, the promise of genomic medicine remains a mostly unfulfilled dream. DNA sequencing has led to significant advances in the molecular sciences and healthcare space, identifying an ever-growing catalog of disease-associated mutations. But our forays into the genome have also highlighted a staggering complexity behind human genetics that goes well beyond the base code of DNA.
Beyond GWAS: Expanding the epigenetic perspective
Large genome-wide association studies (GWAS) have been a mainstay in genetics. By analyzing vast troves of genetic and phenotypic data at a population scale, researchers can identify important associations between genetic variants and specific traits.1,2 The breadth of these studies has continued to grow in recent years and has revealed many thousands of DNA variants that may be linked to disease susceptibility.
But for all their strengths, GWAS are also limited in important ways. Rarely do identified variants occur in protein-coding genes, making it difficult for researchers to understand how these variants exert influence over a given trait.3 One possibility is that intergenic variants contribute to disease pathology by affecting gene expression (rather than protein function) by directly disrupting transcription factor binding or by disturbing the normal epigenetic patterns that govern gene blocks (such as the deletion or addition of CpG sites).4–6 However, investigating these possibilities can be a laborious and time-consuming task, often requiring in-depth and potentially costly follow-up studies for each variant.
Put simply, even as the breadth of GWAS continues to grow, the pace of genetic discovery is not keeping up, partly due to the fundamental lack of context surrounding genetic variants. To unblock the field, researchers need tools that allow them to readily gather more information about each variant, including information about how its epigenetic state impacts the genetic context.
The unseen landscape
Epigenetics was first defined in 1942 by the developmental biologist Conrad Hal Waddington in an attempt to explain the apparent inconsistencies of human genetics.7 Though the mechanics were unclear, Waddington and his colleagues recognized that an organism’s many traits are not only dictated by the contents of its genome but also by how that genome is expressed.
The term “epigenetics” has evolved to hold many related definitions, all generally referring to the array of mechanisms a cell uses to establish stable gene expression patterns without affecting its underlying DNA sequence.4 Histone acetylation, for example, can reduce the chromatin’s bond with DNA, relaxing the chromatin structure and leaving local genes more accessible for expression. Such mechanisms allow cells to selectively activate or silence genes, enabling cells with identical genomes to assume distinct identities.
The status of the epigenome is significantly influenced by a cell’s environment. Factors such as nutrient availability, oxygen levels, mechanical forces and exposure to signaling molecules like cytokines or growth factors can induce specific epigenetic modifications.4, 8 These cues help cells adapt their gene expression profiles dynamically, responding to environmental changes while maintaining long-term stability in critical pathways.
This encompasses both microenvironmental factors (local oxygen levels, for instance) and a person's macroenvironment (prolonged stress, exposure to toxins and smoking). Various stressors and nutrient deficiencies, for example, have been linked to altered epigenetic markers that may have a lasting impact throughout a person’s life.9 Some evidence even suggests that environmental factors like childhood trauma can lead to heritable changes in the epigenome.10
One of the most studied epigenetic marks is cytosine methylation (5mC), especially at CpG sites where a cytosine precedes a guanine.11 Though its effects can vary, DNA methylation (specifically 5mC) typically acts as a repressive mark and serves as a means by which cells dampen or silence gene expression. Of note, CpG sites can cluster at or near genetic control elements where they can influence gene expression.
Methylation patterns are dynamic; however, the process of removing 5mC methylation follows a unidirectional pathway starting with oxidation, specifically to 5-hydroxymethylcytosine (5hmC), which is a semi-stable intermediary, and proceeds through a series of alterations until it is ultimately removed and replaced with unmodified cytosine. The presence of 5hmC thus indicates that a gene’s methylation status is less stable or in an active state of change at the time of sampling.11, 12
The human genome is decorated with a vast and dynamic landscape of 5mC and 5hmC markers that collectively form tissue-specific patterns. But when these patterns are disturbed, either by an underlying mutation or by environmental cues, disease can follow.11, 13–16 Studies show that cancer cells, for instance, often exhibit hypermethylation of tumor suppressor genes, while neurodegenerative diseases like Alzheimer’s involve extensive methylation changes that appear to drive pathology.16, 17 These modifications act as molecular switches, amplifying or silencing genes without immediately altering the underlying DNA sequence. As research advances, it’s increasingly clear that the impact of genetic variants is deeply intertwined with their epigenetic context.
Despite recent progress towards mapping epigenetic interactions, much remains unknown about the ways in which DNA variants, 5mC and 5hmC interact to influence specific disease processes. This gap is largely due to the challenges of studying the genome and epigenome in tandem. Fortunately, the recent advent of 6-base genome sequencing technology may help researchers overcome this challenge and enable a new era of genomic exploration.
Advancing methylation sequencing with 6-base genome sequencing technology
Analyzing both the genome and epigenome in tandem has the potential to greatly improve our understanding of disease mechanics, however, doing so has proven difficult with traditional DNA and methylation sequencing technologies.
Bisulfite sequencing is currently the most common approach to methylation sequencing. This process chemically converts unmethylated cytosines to uracil (and eventually thymine upon sequencing) in a process that can damage the DNA and impact the quality of the sequencing information. With few other options, this approach to studying DNA methylation has allowed the research community to shed new – albeit limited – light on DNA methylation-phenotype associations. But it has come at a cost.
Converting unmethylated cytosines into thymine greatly reduces sequence complexity, making data analysis harder and more time-consuming (due to the difficulty of assembling and aligning these sequences). This method also makes it difficult to distinguish between unmethylated cytosines and a true C > T mutation – the most common mutation in both the mammalian and malignant genomes.18, 19
Additionally, bisulfite sequencing is unable to distinguish between 5mC and 5hmC, leaving out critical details about the stability of methylation patterns. Collectively, this means that it can be very difficult to faithfully identify genetic mutations and the epigenetic marks that surround them.
6-base genome sequencing technology has the potential to overcome these barriers and enable far more accurate and efficient methylation sequencing. This technology leverages a two-nucleotide coding system (as opposed to the standard one nucleotide approach) to identify each base present in the DNA sequence. This method allows the technology to distinguish between a wider variety of bases and reliably distinguish between cytosine, 5mC and 5hmC. This is why it is referred to as “6-base genome sequencing,” because it can annotate the presence of the four traditional bases (A, C, G and T) as well as 5mC and 5hmC. (For more on how 6-base genome sequencing works, an example of the technology is well described in this recent article from Nature Biotechnology.)
Thus far, 6-base genome sequencing has proven to be a highly accurate means of simultaneous DNA and methylation sequencing.
The dawning era of multimodal genetics
More than two decades after the human genome project was completed, we’ve learned that the nuances of gene-phenotype relationships cannot be fully understood if we continue with a narrow focus on DNA. The advent of 6-base genome sequencing empowers researchers to act on that learning by expanding their studies to include both genetic and epigenetic data.
With ready access to multimodal genetic data, researchers can build a higher-resolution understanding of disease processes. The benefits of this can already be seen across a range of applications, from early detection of colorectal cancer to deep tumor characterization using cell free DNA.
In short, the era of DNA sequencing that began with the human genome project may be coming to an end. But in its place comes the era of multimodal genetics, powered in part by key technologies like 6-base genome sequencing.
- Uffelmann E, Huang QQ, Munung NS, et al. Genome-wide association studies. Nat Rev Methods Primers. 2021;1(1). doi: 10.1038/s43586-021-00056-9
- Abdellaoui A, Yengo L, Verweij KJH, Visscher PM. 15 years of GWAS discovery: realizing the promise. Am J Human Genet. 2023;110(2):179-194. doi: 10.1016/j.ajhg.2022.12.011
- Tak YG, Farnham PJ. Making sense of GWAS: using epigenomics and genome engineering to understand the functional relevance of SNPs in non-coding regions of the human genome. Epigenetics & Chromatin. 2015;8(1). doi: 10.1186/s13072-015-0050-4
- Cavalli G, Heard E. Advances in epigenetics link genetics to the environment and disease. Nature. 2019;571(7766):489-499. doi: 10.1038/s41586-019-1411-0
- Hawe JS, Wilson R, Schmid KT, et al. Genetic variation influencing DNA methylation provides insights into molecular mechanisms regulating genomic function. Nature Genetics. 2022;54(1):18-29. doi: 10.1038/s41588-021-00969-x
- Feinberg AP, Levchenko A. Epigenetics as a mediator of plasticity in cancer. Science. 2023;379(6632). doi: 10.1126/science.aaw3835
- Waddington CH. The epigenotype. Int J Epidemiol. 1942;41(1):10-13. doi: doi.org/10.1093/ije/dyr184
- Yang J, Xu J, Wang W, Zhang C, Lei Y, Shi S. Epigenetic regulation in the tumor microenvironment: molecular mechanisms and therapeutic targets. Sig Transduct TargetTher. 2023;8(1). doi: 10.1038/s41392-023-01480-x
- CDC. Epigenetics, health, and disease. Genomics and Your Health. https://www.cdc.gov/genomics-and-health/epigenetics/index.html Published December 2, 2024. Accessed March 12, 2025.
- Tuulari JJ, Matthieu Bourgery, Iversen J, et al. Exposure to childhood maltreatment is associated with specific epigenetic patterns in sperm. Mol Psychiatry. Published online January 3, 2025. doi: https://doi.org/10.1038/s41380-024-02872-3
- Joseph DB, Strand DW, Vezina CM. DNA methylation in development and disease: an overview for prostate researchers. Am J Clin Exp Urol. 2018;6(6):197-218. PMID: 30697577
- Pfeifer GP, Xiong W, Hahn MA, Jin SG. The role of 5-hydroxymethylcytosine in human cancer. Cell Tissue Res. 2014;356(3):631-641. doi: 10.1007/s00441-014-1896-7
- Min JL, Hemani G, Hannon E, et al. Genomic and phenotypic insights from an atlas of genetic effects on DNA methylation. Nat Genet. 2021;53(9):1311-1321. doi: doi.org/10.1038/s41588-021-00923-x
- Do C, Dumont ELP, Salas M, et al. Allele-specific DNA methylation is increased in cancers and its dense mapping in normal plus neoplastic cells increases the yield of disease-associated regulatory SNPs. Genome Biology. 2020;21(1). doi: 10.1186/s13059-020-02059-3
- Davalos V, Lovell CD, Richard Von Itter, et al. An epigenetic switch controls an alternative NR2F2 isoform that unleashes a metastatic program in melanoma. Nat Commun. 2023;14(1). doi: 10.1038/s41467-023-36967-2
- Schaffner SL, Kobor MS. DNA methylation as a mediator of genetic and environmental influences on Parkinson’s disease susceptibility: impacts of alpha-Synuclein, physical activity, and pesticide exposure on the epigenome. Front Genet. 2022;13. doi: 10.3389/fgene.2022.971298
- Wang E, Wang M, Guo L, et al. Genome‐wide methylomic regulation of multiscale gene networks in Alzheimer’s disease. Alzheimer’s & Dementia. 2023;19(8):3472-3495. doi: 10.1002/alz.12969
- Cagan A, Baez-Ortega A, Brzozowska N, et al. Somatic mutation rates scale with lifespan across mammals. Nature. 2022;604(7906):517-524. doi: 10.1038/s41586-022-04618-z
- Iengar P. An analysis of substitution, deletion and insertion mutations in cancer genes. Nucleic Acids Res. 2012;40(14):6401-6413. doi: 10.1093/nar/gks290
- Füllgrabe J, Gosal WS, Creed P, et al. Simultaneous sequencing of genetic and epigenetic bases in DNA. Nat Biotechnol. 2023;41(10):1457-1464. doi: 10.1038/s41587-022-01652-0