The Future Is Bright: Advances and Developments in Flow Cytometry
A range of recent developments are tackling challenges and helping to advance the field of flow cytometry.
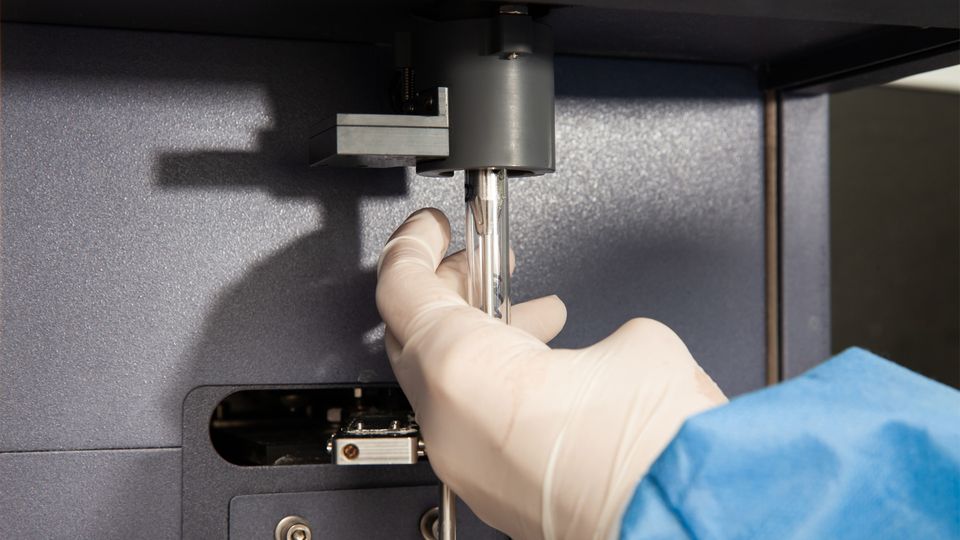
Complete the form below to unlock access to ALL audio articles.
For almost six decades, flow cytometry (FC) has been a cornerstone of clinical and laboratory research, with applications in a wide range of fields, including immune-phenotyping, diagnostics, cell counting and cell cycle analysis.
FC is a powerful tool for multi-parametric cell characterization, even in complex, mixed samples, due to its ability to assess cell properties by light scatter and fluorescence. Cells suspended in a saline solution flow past laser light sources in a single-file manner, known as hydrodynamic focusing.1 When the laser hits the cells, any visible light scattered forwards (forward scatter – FSC) gives information about the cell diameter, while light scattered sideways (side scatter – SSC) provides information about the granularity of the cell.
Further phenotypical characterization of the cell can be achieved with fluorescent dyes or fluorophore-conjugated antibodies. Flow cytometers can also be used for cell sorting, i.e., the physical separation of cells into distinct populations, known as fluorescence-activated cell sorting (FACS).
For many years, FC advances were defined by increasing the number of parameters that could be simultaneously measured, though the fundamental processes of fluorescence-based, polychromatic FC remained largely unchanged.
While early flow cytometers could only analyze one or two parameters, modern fluorescence-based instruments can simultaneously measure up to 30 parameters.2 However, this meant that FC reached a technological ceiling – since each color channel requires its own detector, the number of detectors in a single machine became the limiting factor. Other challenges in traditional FC techniques include relatively high limits of detection, subjective interpretation of results, unstable fluorophores and the need for large numbers of controls.
A range of recent developments are tackling these challenges and helping to advance the field of FC significantly, including spectral FC, nanoparticle cytometry and droplet cytometry. These advances are improving the accuracy and efficiency of FC and opening up new research and clinical applications.
Looking at the full spectral picture: Spectral flow cytometry
In traditional, polychromatic FC, fluorescent light signals are produced when fluorophore-labelled probes are excited by lasers of a corresponding wavelength range. The peak emission spectrum from each fluorophore is isolated by a series of dichroic mirrors and bandpass filters and captured by a single detector.3 As a consequence, the number of detectors available becomes the limiting factor. In addition, as the number of parameters increases, the more individual fluorophores must be used and the closer their emission peaks become, making experiments extremely complex. Spectral cytometry is a rapidly growing technology that captures the full emission spectra of each fluorophore using an array of detectors, overcoming these limitations.4
“It became apparent that there was a physical limitation of about 28–30 colors for polychromatic FC,” says J. Paul Robinson, distinguished professor of cytometry at Purdue University. “However, this is not the case with spectral cytometry. We don’t talk about instruments being restricted by colors, because we don’t yet know the upper limits of how many different colors a spectral cytometer can collect.” Robinson was involved in the development of spectral cytometry technology in the early 2000s, and his lab filed a patent on it in 2004. This patent was then the basis of the first commercially available spectral flow cytometer.
Spectral cytometry captures the emission spectra for each fluorophore across multiple detectors, allowing it to differentiate between fluorochromes with overlapping emission peaks. In order to quantify the signal of each fluorophore, the spectra must be separated, using a spectral unmixing algorithm that fits and identifies individual fluorophores by comparison with single-stained controls.5 One of the benefits of spectral cytometry is that it is less reliant on subjective, manual compensation. “Compensation is a very personal thing, dependent on experience. The math of spectral unmixing is essentially the same as the math of compensation,” explains Robinson. “It’s just performed in an automated manner, which gives it much better consistency and greater accuracy.”
The applications of spectral cytometry have already shown enormous potential. Spectral FC is able to calculate cellular autofluorescence and treat it as another channel. Thus, noise in complex samples is reduced, and identification of rare cell subsets in highly autofluorescent samples such as blood is improved.3 Its role in advancing cancer research has also become apparent, having been used to study subtle changes in immune signatures and characterize complex tumor infiltrates in response to novel treatments.3
Robinson believes that spectral cytometry has even more to offer. “Spectral FC has already achieved around 50 colors in one experiment, and I suspect it will achieve 100 eventually”, he says. “As the numbers of potential parameters increases, we could be able to look at enough biomarkers to predict when a patient’s physiological environment has changed, even before symptoms appear.”
Life in nanoscale: Nanoparticle flow cytometry
FC is instrumental in characterizing cells and continues to enable significant advances across clinical and life sciences. However, the sensitivity limitations of most conventional flow cytometers and assays mean that observations are limited to biomarkers present either intracellularly, or on the surface of cells. Secreted, extracellular biomarkers such as proteins, metabolites and molecules contained in extracellular vesicles (EVs) generally fall below the lower limits of light scatter detection of conventional flow cytometers, with far dimmer signals.6, 7
EVs are nano-sized, cargo-carrying vesicles secreted from cells into the extracellular environment. They are becoming increasingly recognized as both important biomarkers in a range of physiological functions and processes such as aging and the immune response, and for their potential clinical applications.8 Traditional methods of EV analysis such as polymerase chain reaction (PCR) or mass spectrometry can measure the total amount of EV components in a sample, but cannot give a comprehensive picture of the distribution of those components. “These methods are limited in their ability to identify informative biomarkers or bioactive cargo, two key translational goals of EV research,” explains Prof. John Nolan, of the Scintillon Research Institute. Nolan’s lab develops new tools for analysis of EVs, their formation and their interactions with cells – including custom-built nanoparticle flow cytometers along with optimized and validated assays.
“A new generation of more sensitive [FC] instruments and optimized assays with improved specificity and reproducibility make it possible to measure even the smallest EVs and their cargo, down to < 40 nm and 10 or fewer molecules,” he says. However, there are still challenges; even for more sensitive machines, detection efficiencies can vary and create inconsistencies in reporting. To combat this, Nolan is part of a working group developing a framework for standardizing EV FC experiment reporting, known as MIFlowCyt-EV.9 “By designing and optimizing assays that include the proper positive and negative controls, calibrating instruments and reporting results in absolute units of number and brightness, we can address these challenges, enabling results to be compared across instruments and between labs,” explains Nolan. “Standardization is essential for the effective translation of findings from basic research labs into new clinical biomarkers and therapeutics.”
Teaching an old cytometer new tricks: Double droplet cytometry
Although advances in FC technology continue to expand the field, the importance of standard flow cytometers and FACS machines for research advances shouldn’t be overlooked. Droplet microfluidics allows the high-throughput analysis of millions of individual cells or proteins, in volumes as small as 10-15 liters.10 While these droplets and their cargo can be phenotypically sorted, traditional single-layer water-in-oil droplets require custom, technically demanding microfluidics devices, which tend to be slower than standard FACS machines, with limited color channels. Dr. Polly Fordyce, associate professor of bioengineering and genetics at Stanford University, has been developing double emulsion droplet technology. The contents of double emulsion droplets can be phenotyped and sorted using a standard flow cytometer, allowing far higher throughput and multi-parametric analysis.11, 12
“A double emulsion droplet is an aqueous droplet, inside an oil droplet that is surrounded again by an aqueous buffer, similar to a cell membrane,” explains Fordyce. “To a FACS machine, these droplets just look like large cells, so they can be analyzed using a normal flow cytometer.” However, there are still challenges. “We’ve spent a lot of time figuring out how to stabilize the oil–water interfaces, how to keep the droplets in suspension and how to calibrate the FACS machine,” says Fordyce. The Fordyce lab has been using machine learning to characterize the effect of different variables on droplets, to create a model that can predict the droplets produced by given flow rates and surfactants, and then design devices to create droplets with specific parameters.13 “These are all the small things that will push the science forward and make the technology more accessible,” she says.
The double emulsion droplets provide a level of protection from the shear forces of the FC fluidics, allowing examination of more temporal or spatial phenotypes. “The droplet creates a shell around a cell, which enables you to examine secreted proteins that would usually diffuse away. You can encapsulate cell pairs to examine cell–cell interactions or encapsulate enzymes with their substrates to examine enzymatic activity,” explains Fordyce. For Fordyce’s lab, the aim is to use double droplet technology and FC to understand how the sequence of a protein encodes its functions. “Our main goal,” she says, “is pioneering new ways to make thousands to millions of protein variants, and then quantify what they do. For example, understanding how the sequences of enzymes encode the catalytic efficiency, their specificity and the different parts of their enzymatic cycle.” By encapsulating these protein variants in double emulsion droplets and using fluorescent tags, FC and FACS can then be used as a high-throughput methods to sort and identify the active, functional variants. 14
Is the future fluorescent?
Even considering all the recent developments in the field, the advancement of FC shows no signs of slowing down. Robinson believes the next steps for FC may be quantum. “Technology advances to a true generation of quantum cytometers is likely to emerge within 2–3 years”. Quantum measurement could allow the detection of single fluorophores, in turn enabling the identification of extremely rare biomarkers.15 This, combined with single photon detectors, could see the transition of FC from a somewhat subjective, qualitative technology to a fully quantitative technology.
Nolan, too, believes the measurement of single molecules to be a powerful tool for the future of research. “New needs and applications tend to drive the development of new techniques, rather than the other way around.” He says “In EV research, the capabilities of FC will help researchers test hypotheses about how these systems work, and help us to understand, predict and control cells to improve health.”
However, as rapidly as FC advances, we cannot forget about the basic principles that brought us to this point. Novel technologies cannot flourish without labs and institutions investing in the foundations of high-quality FC, and the highly-skilled operators that perform it. “Shared, core facilities with trained operators have been key in turning FC into the powerful tool that it is today,” says Fordyce, “and I hope to see more institutions understanding the value of these central facilities in the future.”
References
1. Picot J, Guerin CL, Van Kim CL, Boulanger CM. Flow cytometry: Retrospective, fundamentals and recent instrumentation. Cytotechnology. 2012;64(2):109-130. doi:10.1007/s10616-011-9415-0
2. Robinson JP, Ostafe R, Iyengar SN, Rajwa B, Fischer R. Flow cytometry: The next revolution. Cells. 12(14):1875. doi:10.3390/cells12141875
3. Novo D. A comparison of spectral unmixing to conventional compensation for the calculation of fluorochrome abundances from flow cytometric data. Cytom Part A. 2022;101(11):885-891. doi:10.1002/cyto.a.24669
4. Nolan JP, Condello D. Spectral flow cytometry. Curr Protoc Cytom. 2013;63(1). doi:10.1002/0471142956.cy0127s63
5. Schmutz S, Valente M, Cumano A, Novault S. Spectral cytometry has unique properties allowing multicolor analysis of cell suspensions isolated from solid tissues. PLoS ONE. 2016;11(8):e0159961. doi:10.1371/journal.pone.0159961
6. Botha J, Pugsley HR, Handberg A. Conventional, high-resolution and imaging flow cytometry: Benchmarking performance in characterisation of extracellular vesicles. Biomedicines. 2021;9(2):124. doi:10.3390/biomedicines9020124
7. Nolan JP. Flow Cytometry of extracellular vesicles: Potential, pitfalls, and prospects. Curr Protoc Cytom. 2015;73(1). doi:10.1002/0471142956.cy1314s73
8. Ibrahim SA, Khan YS. Histology, extracellular vesicles. StatPearls - NCBI Bookshelf. https://www.ncbi.nlm.nih.gov/books/NBK562256/. Published August 14, 2023. Accessed December 11, 2024
9. Welsh JA, Van Der Pol E, Arkesteijn GJA, et al. MIFlowCyt‐EV: A framework for standardized reporting of extracellular vesicle flow cytometry experiments. J Extracell Vesicles. 2020;9(1). doi:10.1080/20013078.2020.1713526
10. Sohrabi S, Kassir N, Moraveji MK. Droplet microfluidics: Fundamentals and its advanced applications. RSC Adv. 2020;10(46):27560-27574. doi:10.1039/d0ra04566g
11. Brower KK, Carswell-Crumpton C, Klemm S, et al. Double emulsion flow cytometry with high-throughput single droplet isolation and nucleic acid recovery. Lab Chip. 2020;20(12):2062-2074. doi:10.1039/d0lc00261e
12. Brower KK, Khariton M, Suzuki PH, et al. Double emulsion picoreactors for high-throughput single-cell encapsulation and phenotyping via FACS. Anal Chem. 2020;92(19):13262-13270. doi:10.1021/acs.analchem.0c02499
13. Lashkaripour A, McIntyre DP, Calhoun SGK, Krauth K, Densmore DM, Fordyce PM. Design automation of microfluidic single and double emulsion droplets with machine learning. Nat Commun. 2024;15(1). doi:10.1038/s41467-023-44068-3
14. Thompson S, Zhang Y, Yang Z, Nichols L, Fordyce PM. FACS‐sortable triple emulsion picoreactors for screening reactions in biphasic environments. Adv Mater Interfaces. Published online December 4, 2024. doi:10.1002/admi.202400403
15. Sabines-Chesterking J, Burenkov IA, Polyakov SV. Quantum measurement enables single biomarker sensitivity in flow cytometry. Sci Rep. 2024;14(1). doi:10.1038/s41598-023-49145-7